Dickinson REC 2024 Annual Report
((Research Report, Dickinson REC, December 2024))Dickinson Research Extension Center Annual Report 2024
Chris Augustin, Glenn Martin, Llewellyn L. Manske, Doug Lanblom, Victor Gomes, Krishna Katuwal, and Rutendo Nyamusamba
2024 Annual Report
Current Staff-2024
Chris Augustin Director/Soil Scientist
Victor Gomes Extension Cropping Systems Specialist
Cristin Heidecker Administrative Secretary
Krishna Katuwal Research Agronomist
Doug Landblom Associate Research Extension Center Specialist
Llewellyn Manske Scientist of Rangeland Research
Glenn Martin Research Specialist
Dean Nelson Ag Research Technician
Rutendo Nyamusamba Extension Conservation Agronomist Specialist
Phyllis Okland Administrative Assistant
Samuel Olorunkoya Ag Research Tech
Garry Ottmar Livestock Research Specialist
Wanda Ottmar Research Technician
Sheri Schneider Information Processing Specialist
Michael Strode Computer Technician
Lee Tisor Research Specialist
2024 Seasonal/Temporary Employees | |||
John Urban | Michele Stoltz | Chuck Wanner | Tom Grey |
Frederick Nortje | Anastasia Kempenich | Mahmuda Rahman | Keaton Meek |
Miguelangel Torres | Javier Lugo |
Advisory Board
Jacob Odermann-Chair
Dustin Elkins
John Hendrickson
Ryan Kadrmas
Bill Kessel
Kevin Kessel
Mike Gerbig
Chip Poland
Levy Steiner
Edward Cuskelly
Blake Johnson
NDSU Dickinson Research Extension Center 1041 State Avenue
Dickinson, ND 58601
Phone: (701) 456-1100
Fax. (701) 456-1199
Website: https://www.ndsu.edu/agriculture/ag-hub/research-extension-centers-recs/dickinson-rec Email: NDSU.Dickinson.REC@ndsu.edu
NDSU is a equal opportunity institute.
NDSU does not discriminate in its programs and activities on the basis of age, color, gender expression/identity, genetic information, marital status, national origin, participation in lawful off-campus activity, physical or mental disability, pregnancy, public assistance status, race, religion, sex, sexual orientation, spousal relationship to current employee, or veteran status, as applicable. Direct inquiries to Vice Provost for Title IX/ADA Coordinator, Old Main 201, NDSU Main Campus, 701-231-7708, ndsu.eoaa@ndsu.edu. This publication will be made available in alternative formats for people with disabilities upon request, 701-231-7881.
2024 Variety Trials
Winter Wheat........................................................................................................................ 5
Barley................................................................................................................................... 6
Durum................................................................................................................................... 7
Field Pea............................................................................................................................... 8
Flax....................................................................................................................................... 9
Barley-Glen Ullin................................................................................................................. 10
Spring Wheat-Glen Ullin...................................................................................................... 11
Hard Red Spring Wheat........................................................................................................ 12
Oat...................................................................................................................................... 13
Organic Hard Red Spring Wheat........................................................................................... 14
Grassland Research
Increasing Organic Carbon in Northern Plains Grassland Soils................................................ 15
Proactive Preventive Pestiferous Grasshopper Habitat Management........................................ 23
Agronomy Research
Soybean Phosphorus Impacts on Spring Wheat...................................................................... 53
Sulfur Impacts on Spring Wheat............................................................................................ 55
Canola Sulfur Impacts on Spring Wheat................................................................................ 56
Boron Impacts on Canola...................................................................................................... 57
Phosphorus Impacts on Soybean Grown in Southwest North Dakota....................................... 58
Crop and Pest Reports by Victor Gomes................................................................................ 60
Planting cover crops still beneficial in fall, even under dry conditions...................................... 68
New program offers incentives for adoption of cover crops..................................................... 70
Sulfur Fertilizer for Canola Production in Southwest North Dakota......................................... 71
Livestock Research
Integrated Systems Single Timed-AI Heifer Development and
Non-Pregnant Heifer Finishing Profitability.................................................................. 73
The Value of Alfalfa for Nutrient Recycling in a Diverse Crop Rotation........................ 77
Effect of Drought and Subsequent Precipitation (2016-2020) on Soil pH, Microbial Biomass,
and Plant Nutrient Changes in the Semi-Arid Region of Western North Dakota, USA..... 79
Integrated Systems Outreach Programs Prepared and Hosted by the Dickinson Research
Extension Center......................................................................................................... 80
DREC Field Days and Cover Crops Field Days...................................................................... 84
DREC Outreach
Outreach List 2024............................................................................................................... 86
Outreach Collaborators and Grants 2024................................................................................ 89
2024 Outreach List for DREC............................................................................................... 90
2024 Weekly Updates........................................................................................................... 91
Weather Summary................................................................................................................ 93
2024 Variety Trials
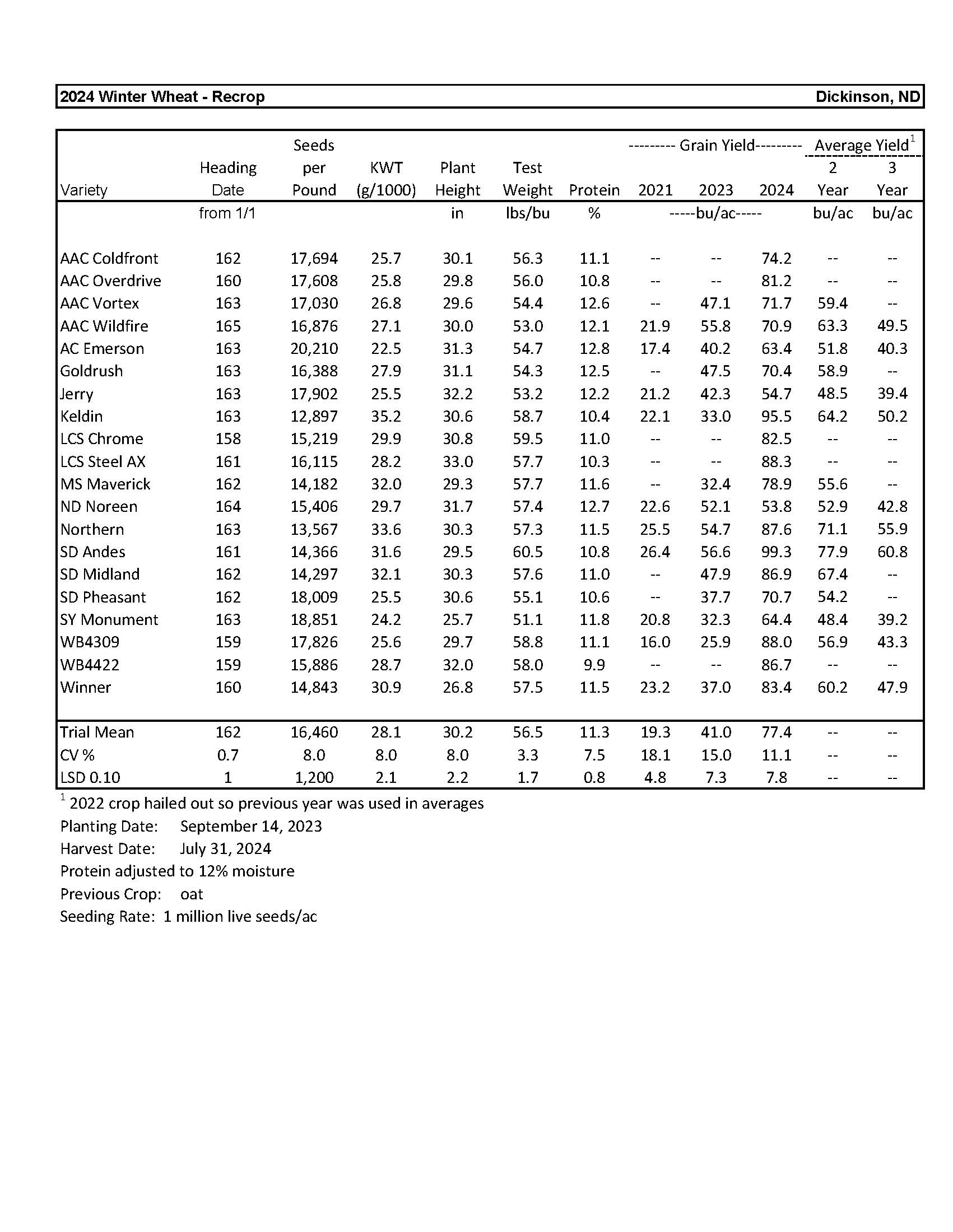
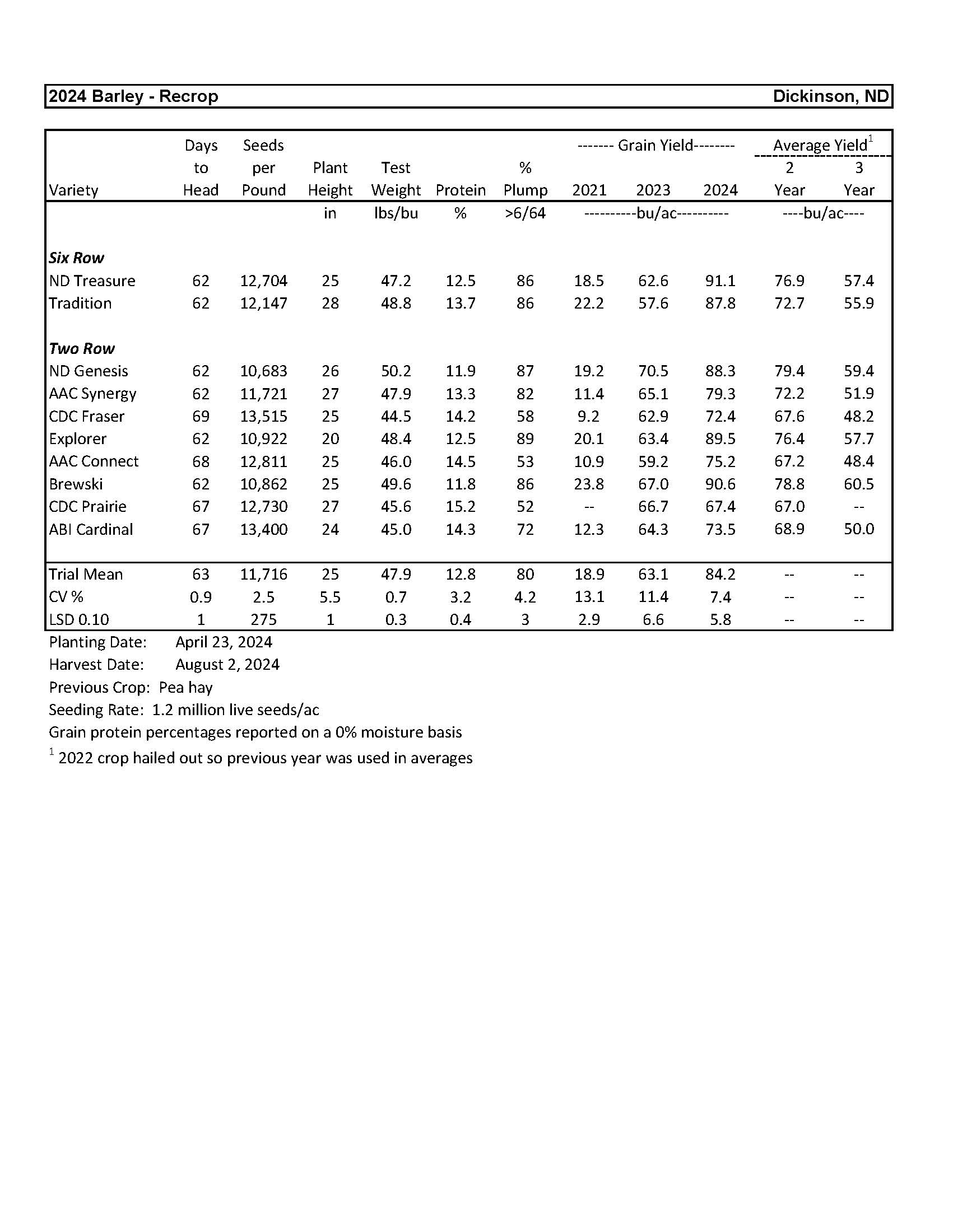
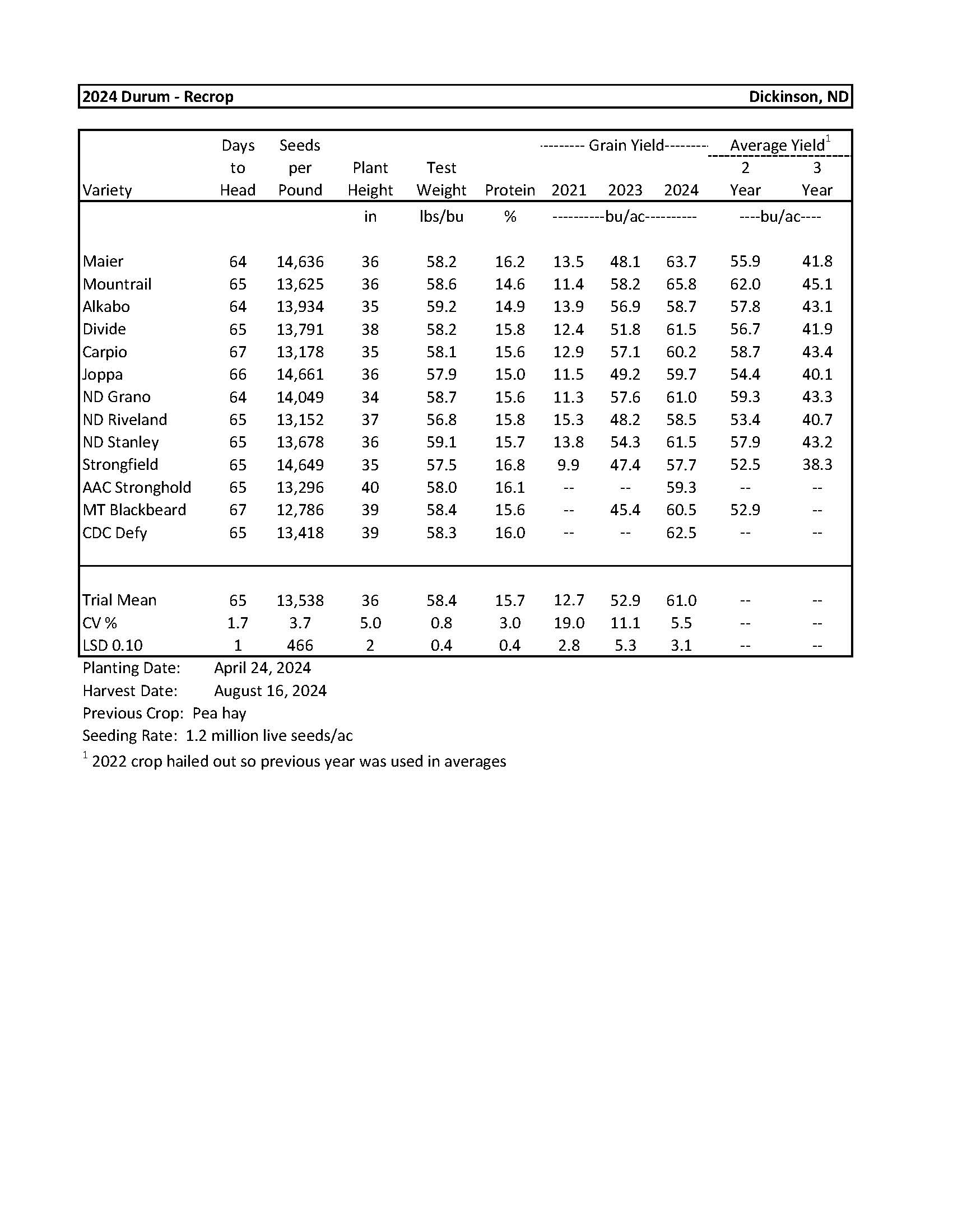
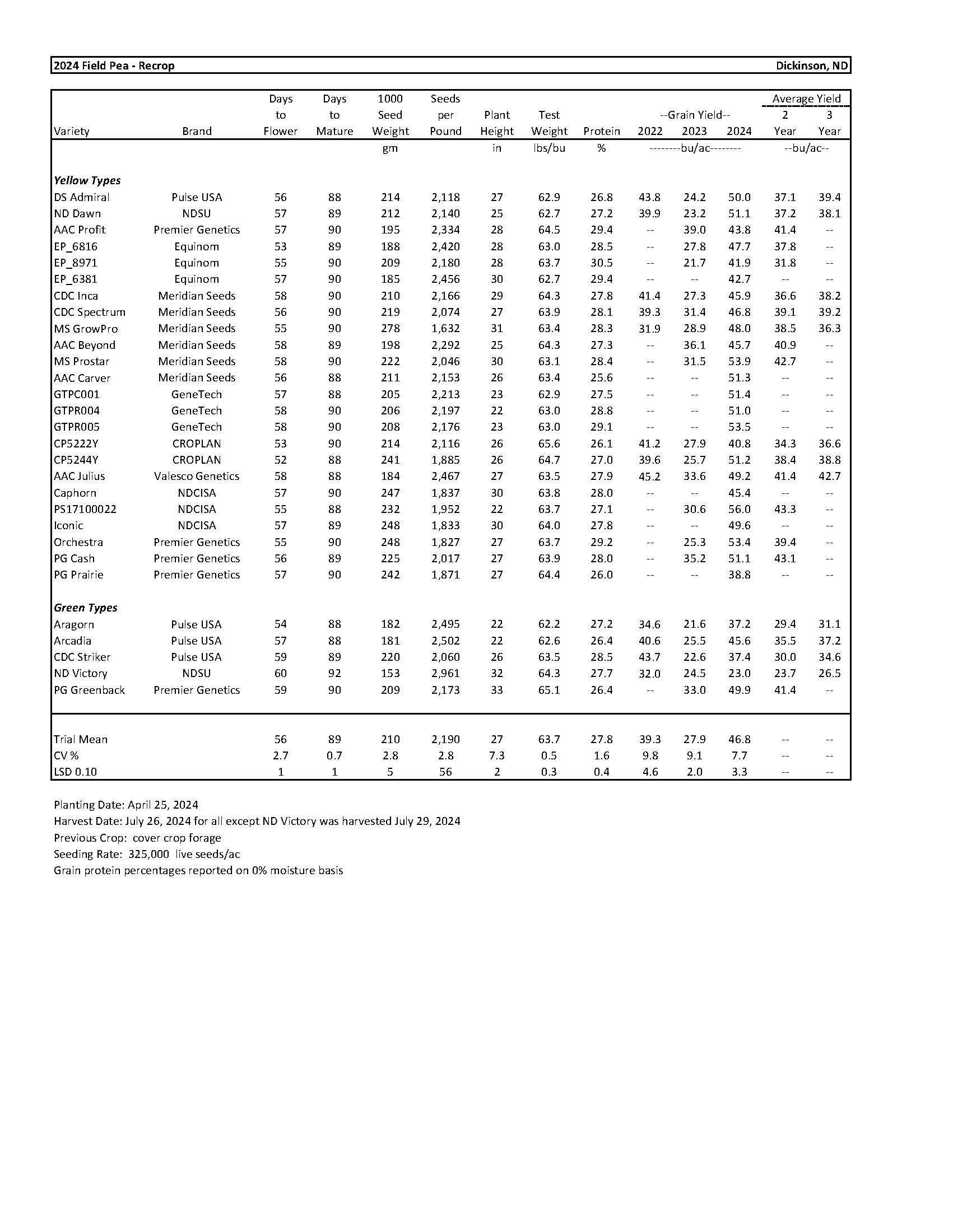
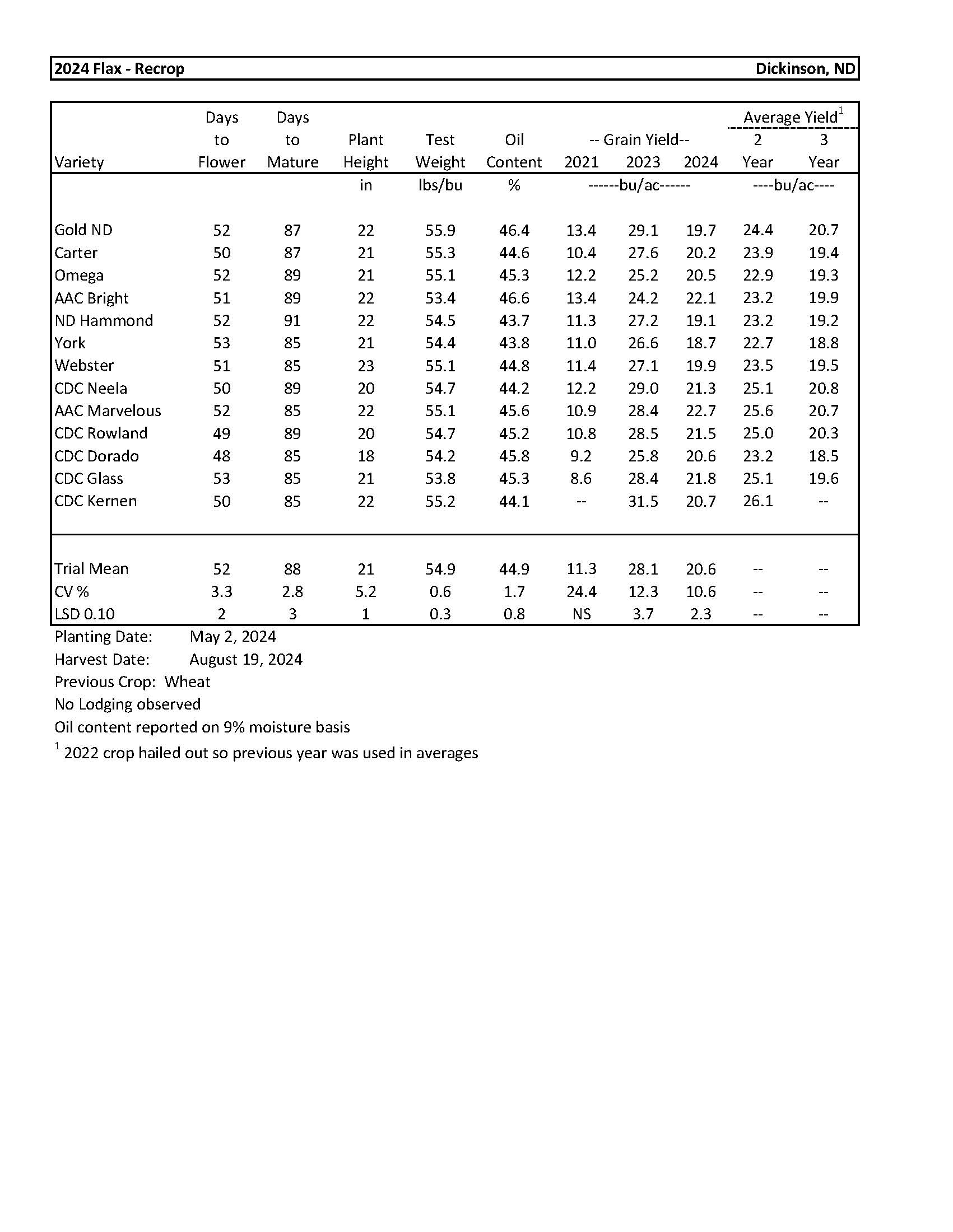
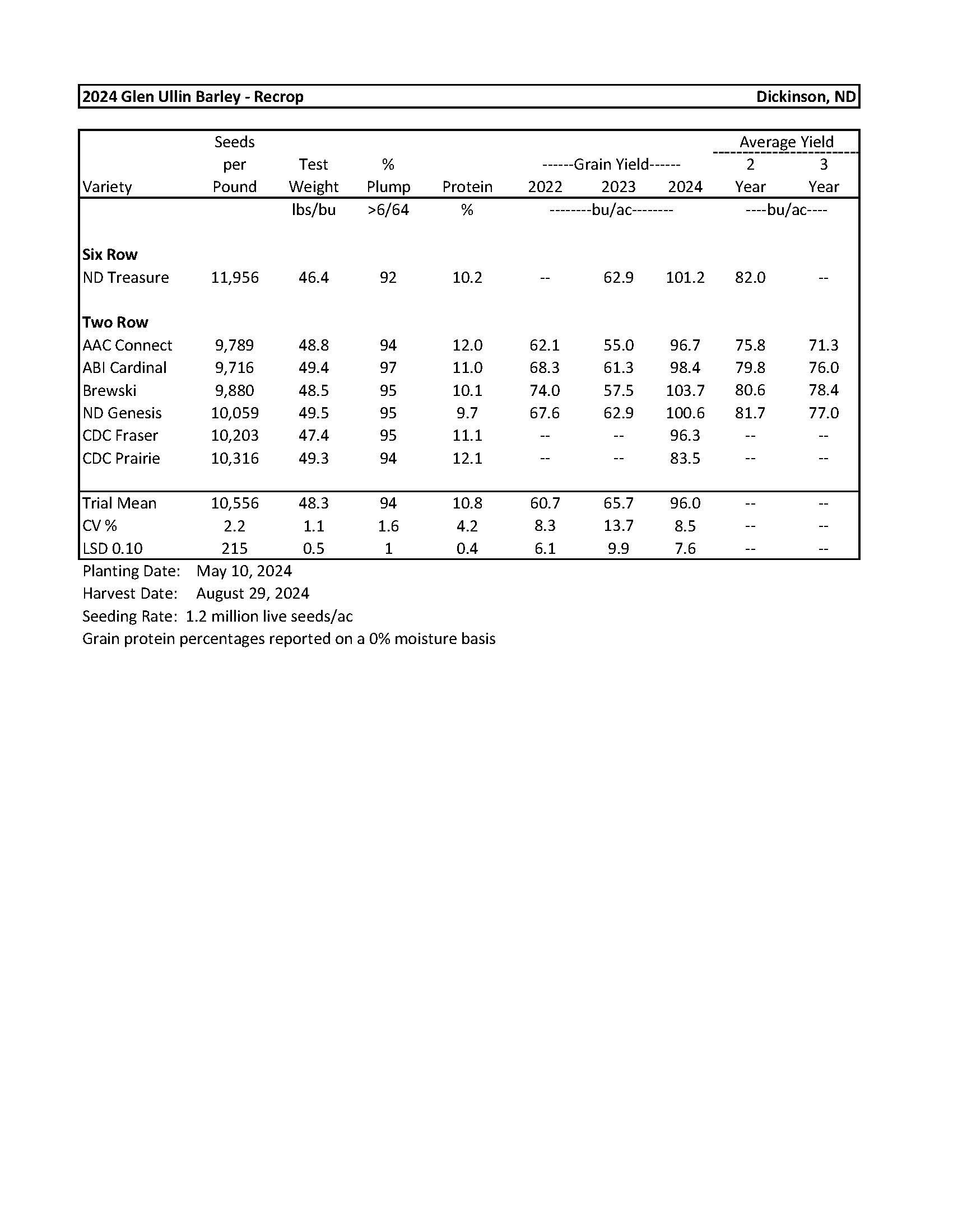
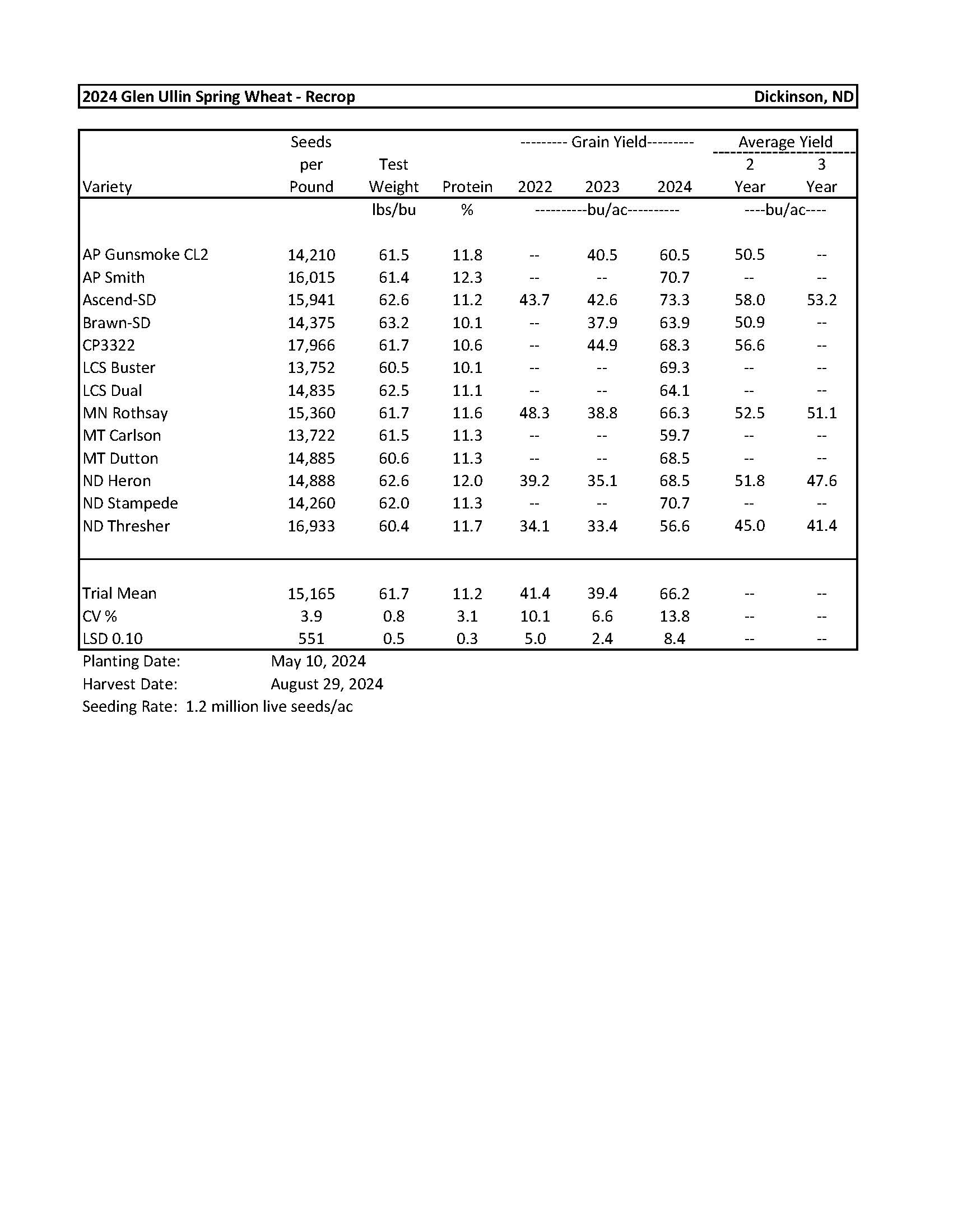
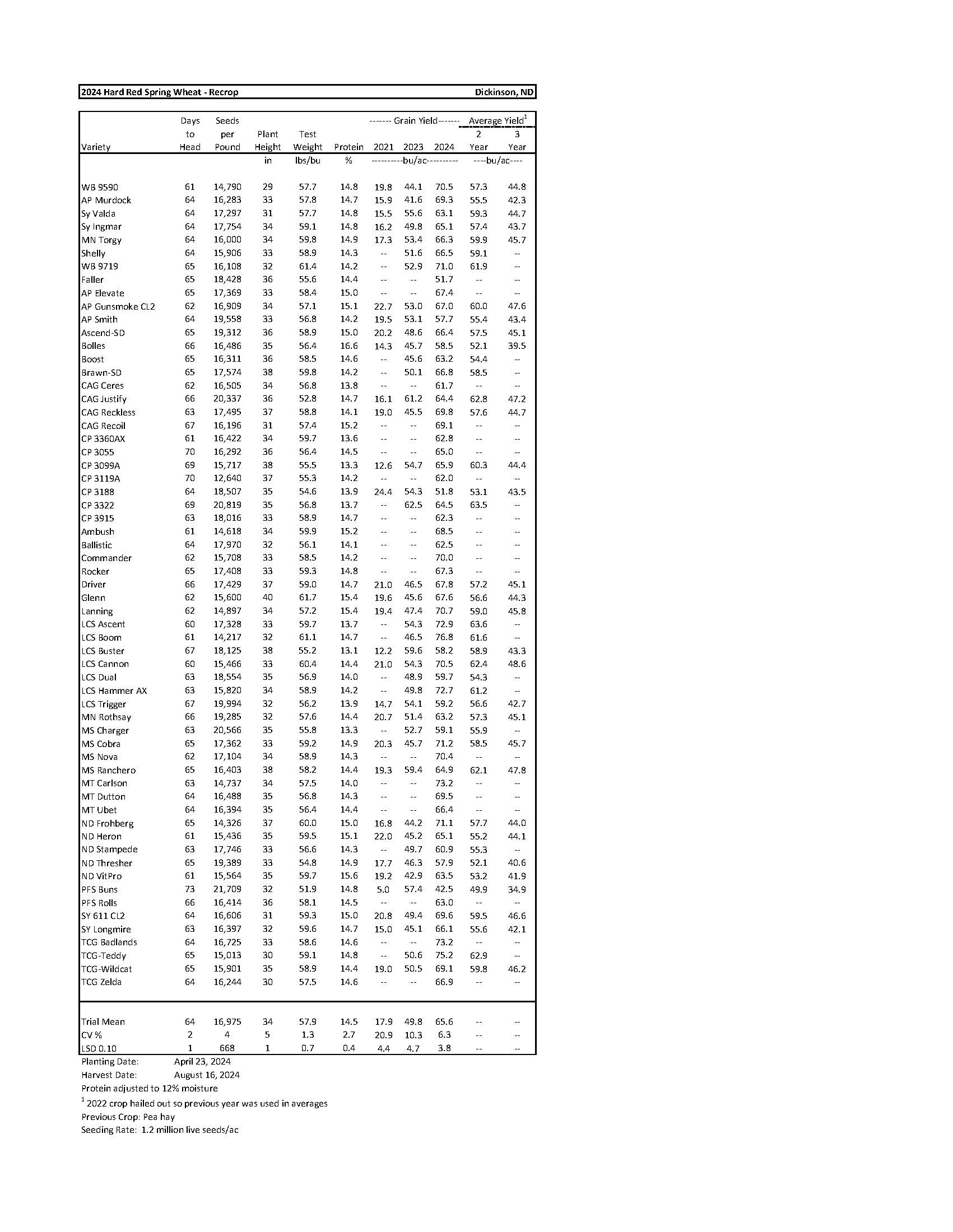
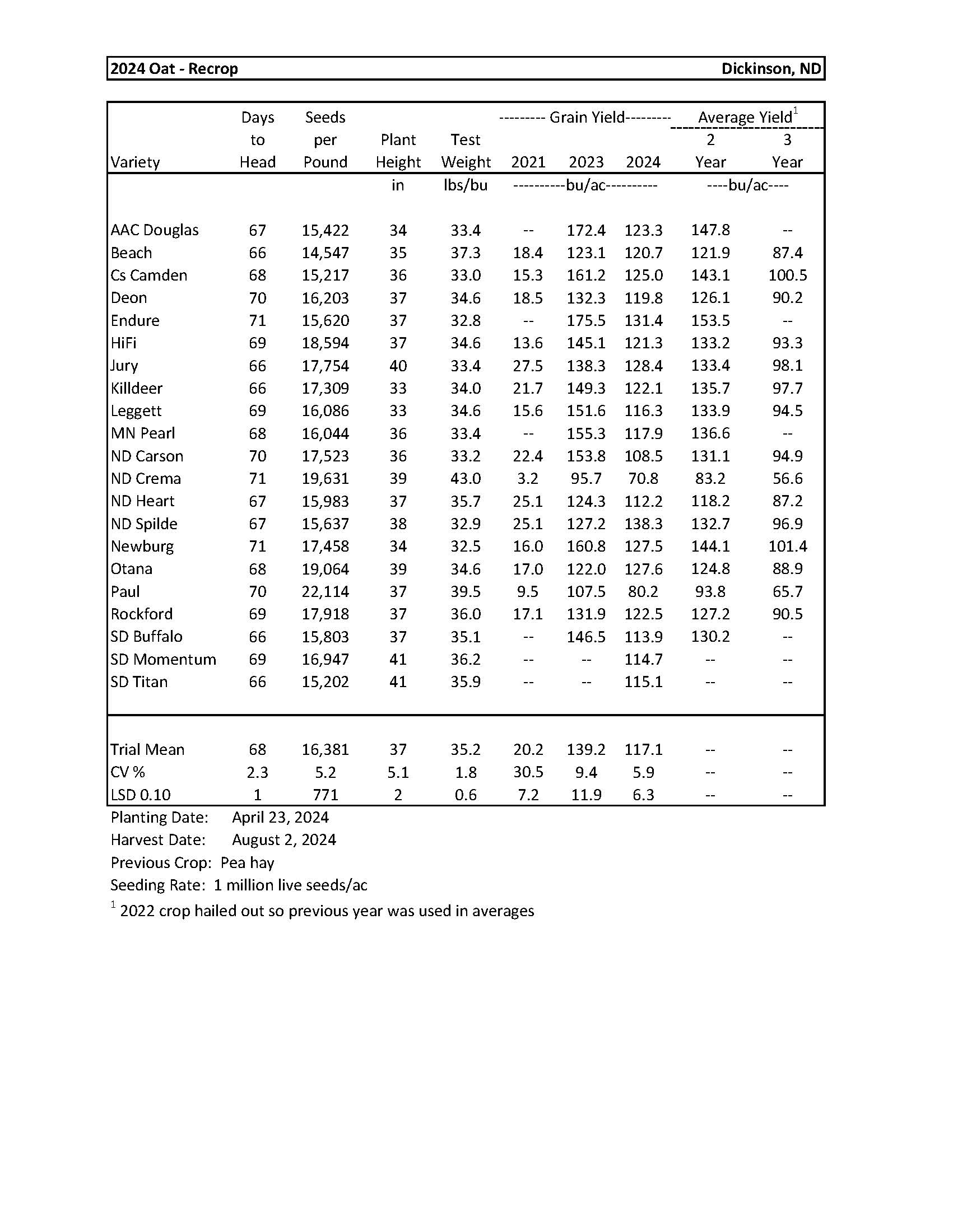
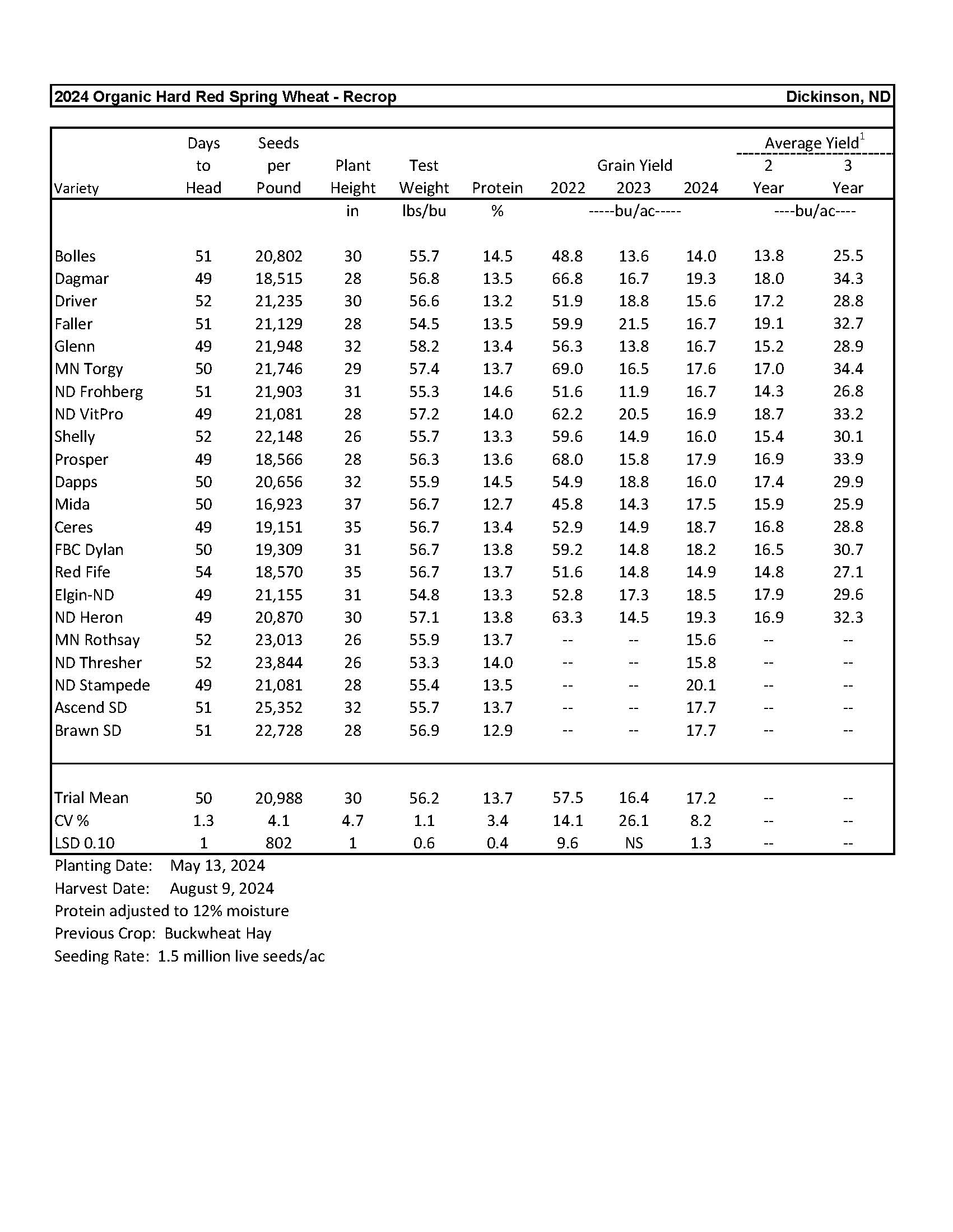
Grassland Research
Increasing Organic Carbon in Northern Plains Grassland Soils
Llewellyn L. Manske PhD
Scientist of Rangeland Research
North Dakota State University
Dickinson Research Extension Center
DREC 24-5007b1
Carbon is the fourth most abundant element in the universe by mass. Carbon based compounds form the basis of all life on earth. Carbon can form more different compounds than all other elements combined. The carbon cycle is a major biogeochemical cycle in which carbon is exchanged among the atmosphere, plants, and soil. Autotrophic plants use solar energy, atmospheric carbon dioxide plus water to produce carbohydrates and oxygen. Heterotrophic organisms depend on photosynthetic carbohydrates as a source of energy and other carbon compounds. Carbon is a major essential element required for life to be maintained (Anonymous 2023, FAO 2023).
Carbon as carbon dioxide in the atmosphere is a vital greenhouse gas that has for 10,000 years been providing a constant warm normal global temperature in which some water is liquid and life as we know can persist (Anonymous 2023). Atmospheric carbon dioxide reradiates some of earth’s escaping longwave infrared energy in order to maintain a stable global temperature (Working Group I 2007).
However, since the start of the industrial revolution in 1750, the quantity of carbon in the atmosphere has increased above the optimal level which is causing greater quantities of longwave infrared energy to be returned back to earth causing the mean global temperature to increase (Working Group I 2007).
The source of the increased carbon emissions in the atmosphere is from the extraction and burning of geologic fossil carbon for fuel (Rosen 2021). After 270 years, the additional carbon in the atmosphere has increased the mean global temperature by about 1.2° C (2.16° F) (Rosen 2021).
The amount of carbon on earth is effectively constant. The deep earth contains about 2.039 billion billion tons of carbon with about 66% of that in the core. The oceans contain about 44,092 billion tons of carbon. Geologic fossil fuels (coal, oil, and natural gas) consist of about 4,409 billion tons of carbon. The terrestrial soils contain about 1,764 billion tons of carbon. The atmosphere consists of about 1,006 billion tons of carbon. The biosphere consisting of all terrestrial plants and animals contain about 672 million tons of carbon. The earth’s soils contain more carbon than the atmosphere and all the plants and animals combined (Anonymous 2022, 2023).
The soils of grasslands are storing large quantities of carbon but are capable of holding much more carbon than they currently do (FAO 2023). A substantial increase in terrestrial soil organic carbon from atmospheric carbon dioxide captured and fixed during plant photosynthesis can be as effective at reducing the rate of global warming as the primary objective to reduce emissions of carbon dioxide from fossil fuel burning (FAO 2017).
During the eighteen year period of 1990 to 2007, terrestrial soil organic carbon storage increased at a rate of 2.76 billion tons C/yr (Schimel et al. 2015). During the eleven year period of 2007 to 2017, terrestrial soil organic carbon storage increased at a rate of 3.98 billion tons C/yr (Keenan and Williams 2018). The increases in the quantities of atmospheric carbon dioxide have improved the efficiencies of plant photosynthetic processes resulting in increased plant biomass growth and thus greater dead plant material entering the soil and increasing the quantity of soil organic carbon.
The increase in plant biomass production resulting from the increase of atmospheric carbon dioxide causing an improved efficiency in photosynthesis can be enhanced on grasslands by increasing the quantity of available mineral nitrogen, by increasing the biomass of rhizosphere microorganisms, by increasing the quantity of exudated short carbon energy from the surplus fixed carbon produced by grass lead tillers during vegetative growth stages, by removing 25% to 33% of the aboveground leaf biomass with partial defoliation by grazing livestock during the period of 1 June to 15 July (Manske 2018).
The increase of atmospheric carbon dioxide plus the increase in available mineral nitrogen will result in a greater increase in grass herbage biomass that will permit a 40% increase in stocking rate above the standard seasonlong stocking rate for the particular pasture soil types.
The greater the increase in grass herbage biomass production, the greater the increase in the quantity of soil organic carbon.
Perennial grasses consist of 44% carbon, 40% oxygen, 8% hydrogen, and 8% minerals (Manske 2013).
The source of soil organic carbon in grasslands is dead plant and soil microbial material which originated from atmospheric CO2 captured and fixed during plant photosynthesis, and additionally, from the deposits of manure and urine by grazing livestock.
Greater quantities of soil organic carbon improve the physical and chemical properties of soil and soil health, by increasing nutrient storage and availability, increasing cation exchange capacity, increasing particle aggregation, improving soil structure and stability, and increasing water holding capacity (FAO and ITPS 2015).
Efforts to increase the quantities of soil organic carbon in grasslands through implementation of biologically effective management will cause extensive improvement in ecosystem functionality and great increases in grass herbage biomass production which results in the increase in economic wealth captured per acre. These ecosystem improvements and the resulting reductions of atmospheric CO2 are sufficient enough by themselves to justify the needed changes in management practices.
Soil organic carbon is usually categorized into three pools: a labile pool, an intermediate pool, and a refractory (stable) pool. The concept of organic carbon pools are used for improving understanding of SOC dynamics. The quantity of carbon allocated to each pool cannot be measured separately. The labile pool includes easily degradable plant material, microbial biomass, and labile metabolites, and may turn over within a few months or years. The intermediate pool comprises microbially processed organic matter that is partially stabilized on mineral surfaces which also may be protected within aggregates, with turn over times in the range of decades. The refractory pool includes the highly stabilized organic matter and mineral complexes that may remain in soils for centuries or millennia (FAO and ITPS 2015).
Long term stabilization of soil organic carbon requires action from microorganisms and occurs from the interaction of reactive mineral surface area with decomposed products derived by microbial enzymatic breakdown and metabolism forming a surface binding within microaggregates which reduces exposure to additional enzymatic degradation (FAO and ITPS 2015).
Soils do have some inorganic carbon (SIC) as carbonates and hydrogencarbonates (bicarbonate), which have little importance in the biogeochemical processes within a grassland ecosystem (FAO and ITPS 2015).
The quantity of soil organic carbon is dynamic, determined by the balance of carbon material entering the soil as organic residue, and the amount leaving the soil as CO2 through microbial decomposition and respiration. Organic carbon material must be continually added as annual input to the soil (FAO 2017).
The quantity of carbon respired from soils was derived from decomposition of soil organic matter input by soil microbes and was approximately balanced with the quantity of atmospheric CO2 captured and fixed by the annual net primary production of plant biomass (Schimel et al. 1997).
The CO2 released by burning geologic fossil fuels contain carbon with different isotopic ratios than the carbon from plant residue decomposed and released from soils by microbial respiration as CO2 (Anonymous 2023).
The annual quantity of CO2 released from soils to the atmosphere is about ten times larger than the annual quantity of CO2 released to the atmosphere by fossil fuel burning (Schimel 1995). As a result of the improvements in the efficiencies of plant photosynthetic processes, the increases in the quantities of atmospheric carbon dioxide removal, and the increases in the quantities of terrestrial soil carbon storage now occur at a rate equivalent to all of the CO2 emissions from the soils plus about 33.7% of the CO2 emissions from all the fossil fuel burning per year. This is the reason, the rate of global warming is increasing at a rate slower than expected (Schimel et al. 2015, Keenan and Williams 2018).
Nutrient cycling and availability has superior effectiveness and improved efficiencies in highly functional grasslands with soils containing greater quantities of organic carbon (FAO and ITPS 2015). Most of the nutrients consumed and used by livestock for maintenance are returned to the ecosystem in feces and urine. About 15% of the nutrients contained in the consumed leaf material is extracted by stocker heifers and steers and retained for growth. About 30% of the nutrients contained in the consumed leaf material is extracted by lactating cows, with a portion retained by the cow for production, and the remainder of the extracted nutrients passed to her calf for growth (Russelle 1992, Gibson 2009).
During the growing season, a 1200 pound lactating cow consumes 915 lbs forage per month, (44% C) 403 lbs per month is carbon, (25-40%, mean 33%) 133 lbs per month is nondigestible carbon and returned to the soil through feces and urine (FAO 2023), (67%) 270 lbs of carbon per month is digestible, with (30%) 81 lbs of carbon per month used by cow and calf for growth and the remainder used for maintenance.
Nearly all of the essential elements used in the annual production of plant herbage biomass, soil organism biomass, insect biomass, and wildlife biomass are retained and recycled within the grassland ecosystem and only a small portion of the essential elements are removed by livestock as growth in weight (Manske 2013). Fully functional grassland ecosystems with large quantities of stored soil organic carbon are capable of capturing greater quantities of carbon, hydrogen, nitrogen, and oxygen than the amounts lost or removed annually.
Northern Plains grassland ecosystems are perpetually sustainable with the implementation of biologically effective grazing management strategies that activate all ecosystem biogeochemical processes and all grass plant internal physiological grass growth mechanisms to function at biologically potential levels (Manske 2018).
Soil Organic Carbon Project
A project was started in 2022 to quantitatively determine the amount of soil organic carbon accumulation differences in Northern Plains native mixed grass prairie soils managed by three treatments.
Study Area
The native rangeland study sites were on the Dickinson Research Extension Center (DREC) ranch, located in Dunn County, 20 miles north of Dickinson, in western North Dakota.
Long-term mean annual temperature was 42.2° F (5.7° C). January was the coldest month, with a temperature of 14.9° F (-9.5° C). July and August were the warmest months, with mean temperatures of 69.7° F (20.9° C) and 68.5° F (20.3° C), respectively. Long-term mean annual precipitation was 17.1 inches (434.3 mm). The amount of precipitation received during the perennial plant growing season (April to October) was 14.5 inches (367.3 mm) and was 84.6% of the annual precipitation (Manske 2024).
Soils were primarily Typic Haploborolls. The native rangeland vegetation was the Wheatgrass-Needlegrass Type (Barker and Whitman 1988, Shiflet 1994) of the mixed grass prairie. The dominant native range grasses were western wheatgrass (Pascopyrum smithii), needle and thread (Hesperostipa comata), blue grama (Bouteloua gracilis), and threadleaf sedge (Carex filifolia).
Management Treatments
Three management treatments were initiated in 1983 and have been evaluated for 40 years in 2022 (1) long-term nongrazed control, (2) traditional sesonlong, and (3) twice-over rotation system.
The long-term nongrazed control management treatment was designed with two large replicated exclosures and had not been grazed, mowed, or burned for more than 30 years before initiation.
The traditional seasonlong treatment was designed with two replicated pastures. Each pasture was grazed for 137 days from early June to mid October stocked at 2.86 acres per cow-calf pair per month.
The twice-over rotation grazing system was designed with two replicated systems each with three pastures. Each pasture was grazed two times per growing season, 15 days the 1st rotation and 30 days the 2nd rotation plus 2 days for compensation for rotation dates on weekends. Each system was grazed 137 days from early June to mid October stocked at 2.20 acres per cow-calf pair per month.
Procedure
Soil organic carbon (SOC) was determined from four replicated soil core samples collected at silty ecological sites from protected exclosures for the nongrazed treatment, and from grazed sites for the seasonlong and twice-over rotation treatments with the 1 inch Veihmeyer soil tube at incremental depths of 0-6, 6-12, 12-24 inches on monthly periods during May, June, and July. Analysis of soil core samples for total organic carbon was conducted at the North Dakota State University Soil Testing Laboratory.
Results
Growing Season Precipitation
The perennial plant growing season is 6 months from mid April to mid October. During 2022, precipitation in May, June, and July was at 3.17, 2.02, and 3.71 inches respectively, with no water deficiencies. Total growing season precipitation was 16.11 inches, 111.8% of LTM, with two months, August and September with water deficiency conditions.
During 2023 precipitation in May, June, and July was 2.69, 1.91, and 2.21 inches, respectively, with no water deficiencies. Total growing season precipitation was 12.92 inches, 89.67% of LTM, with zero months with water deficiency conditions (Manske 2024).
Global Soil Carbon in Grasslands
The Food and Agriculture Organization (FAO) of the United Nations completed its Global Assessment of Soil Carbon in Grasslands in early 2023. The most similar category to the Northern Plains was the improved natural grasslands of North America which included the more productive native grasslands from the combined area of north and south mixed grass prairie minus the high sand and high clay sites. The southern mixed grass prairie has higher mean temperatures and greater rates of evapotranspiration resulting in lower quantities of stored SOC then the northern mixed grass prairie and the mean combined data from both the southern and northern mixed grass prairie would tend to be lower than data just from the northern mixed grass prairie.
The mean quantity of stored SOC from the combined southern and northern mixed grass prairie to a 30 cm (12 in) depth was 26.76 tons C/ac with a current mean annual input of 1.1 tons C/ac/yr and has a mean minimum quantity of annual input needed to maintain the current storage level was 0.62 tons C/ac/yr (FAO 2023).
The FAO carbon sample protocol uses the soil depth to 30 cm (12 in) because about 66% of all the SOC is usually located in the top foot of the soil column. The second foot contains about 33% of the organic carbon. Additional carbon can be located at deeper depths, as deep as the soil organisms descend. The quantity below the second foot contributes very little change to the total amount. Usually, the greatest changes in SOC, gains or losses, occur in the second foot. The top foot is usually more stable.
The rate that stored SOC is deposited or removed (used) from each increment of soil depth varies from month to month and year to year. The rhizosphere soil microorganism activity that is responsible for production and movement of stored SOC is strongly influenced by the changes in temperature, precipitation, and the rate of evapotranspiration, except that, their rate of activity does not change rapidly and does not show immediate change in activity as a response to a single environmental event.
Stored SOC as effected by management treatment
The current FAO data on quantity of stored SOC by regional grasslands is a valuable standard from which to compare this studies’ data from the Northern Plains mixed grass prairie.
The FAO standard data from the combined south and north mixed grass prairie to a 30 cm (12 in) soil depth was 26.76 tons C/ac, with annual input of 1.1 tons C/ac.
The stored SOC to a 30 cm depth on the nongrazed treatment in 2022 was 33.25 tons C/ac which was 24.3% greater than the FAO data. In 2023, the SOC from the nongrazed treatment was 32.79 tons C/ac (a small reduction) which was 22.5% greater than the FAO data (table 3), with an annual input of 0.80 tons C/ac.
The stored SOC to a 30 cm depth on the seasonlong treatment in 2022 was 47.90 tons C/ac which was 79.0% greater than the FAO data. In 2023, the SOC from the seasonlong treatment was 56.77 tons C/ac which was 112.1% greater than the FAO date (table 3), with an annual input of 1.38 tons C/ac.
The stored SOC to a 30 cm depth on the twice-over treatment in 2022 was 64.34 tons C/ac which was 140.4% greater than the FAO data. In 2023, the SOC from the twice-over treatment was 68.51 tons C/ac which was 156.0% greater than the FAO data (table 3), with an annual input of 1.67 tons C/ac for the top foot of soil.
Acknowledgment
I am grateful to Sheri Schneider for assistance in production of this manuscript and for development of the tables. I am grateful to John Urban for assistance in this project for field data collection.
Table 1. Total organic carbon at two soil depths and at three dates as a percentage, pounds per acre, and tons per acre from three treatments, twice-over, seasonlong, and nongrazed, 2022. | |||||||||
---|---|---|---|---|---|---|---|---|---|
15 May | 15 Jun | 15 Jul | |||||||
Soil Depth Inches |
OC% |
lbs/ac |
Tons/ac |
OC% |
lbs/ac |
Tons/ac |
OC% |
lbs/ac |
Tons/ac |
Twice-over | |||||||||
0-6 | 5.83 | 91,161.79 | 45.58 | 4.91 | 76,776.05 | 38.39 | 5.20 | 81,310.69 | 40.66 |
6-12 | 2.90 | 51,261.09 | 25.63 | 2.14 | 37,827.15 | 18.91 | 2.70 | 47,725.84 | 23.86 |
0-12 | 4.28 | 142,422.88 | 71.21 | 3.44 | 114,603.20 | 57.30 | 3.87 | 129,036.53 | 64.52 |
Seasonlong |
|
|
|
|
|
|
|
|
|
0-6 | 4.22 | 65,986.75 | 32.99 | 3.99 | 62,390.32 | 31.20 | 3.85 | 60,201.18 | 30.10 |
6-12 | 2.17 | 38,357.43 | 19.18 | 1.65 | 29,165.79 | 14.58 | 1.77 | 31,286.94 | 15.64 |
0-12 | 3.13 | 104,344.18 | 52.17 | 2.75 | 91,556.11 | 45.78 | 2.75 | 91,488.12 | 45.74 |
Nongrazed |
|
|
|
|
|
|
|
|
|
0-6 | 2.57 | 40,186.24 | 20.09 | 2.49 | 38,935.31 | 19.47 | 2.51 | 39,248.04 | 19.62 |
6-12 | 1.75 | 30,933.41 | 15.47 | 1.48 | 26,160.83 | 13.08 | 1.36 | 24,039.68 | 12.02
|
0-12 | 2.13 | 71,119.65 | 35.56 | 1.95 | 65,096.14 | 32.55 | 1.90 | 63,287.72 | 31.64 |
Table 2. Total organic carbon at two soil depths and at three dates as a percentage, pounds per acre, and tons per acre from three treatments, twice-over, seasonlong, and nongrazed, 2023. | |||||||||
---|---|---|---|---|---|---|---|---|---|
15 May | 15 Jun | 15 Jul | |||||||
Depth Inches |
OC% |
lbs/ac |
Tons/ac |
OC% |
lbs/ac |
Tons/ac |
OC% |
lbs/ac |
Tons/ac |
Twice-over | |||||||||
0-6 | 6.65 | 103,983.86 | 51.99 | 4.75 | 74,274.19 | 37.14 | 4.15 | 64,892.18 | 32.45 |
6-12 | 3.85 | 68,053.51 | 34.03 | 3.35 | 59,215.39 | 29.61 | 2.30 | 40,655.34 | 20.33 |
0-12 | 5.16 | 172,039.37 | 86.02 | 4.01 | 133,484.58 | 66.74 | 3.17 | 105,547.52 | 52.77 |
Seasonlong |
|
|
|
|
|
|
|
|
|
0-6 | 5.00 | 78,183.35 | 39.09 | 4.90 | 76,619.69 | 38.31 | 4.65 | 72,710.52 | 36.36 |
6-12 | 2.75 | 48,609.65 | 24.30 | 2.15 | 38,003.91 | 19.00 | 1.50 | 26,514.35 | 13.26 |
0-12 | 3.81 | 126,793.00 | 63.40 | 3.44 | 114,623.36 | 57.31 | 2.98 | 99,224.87 | 49.61 |
Nongrazed |
|
|
|
|
|
|
|
|
|
0-6 | 3.15 | 49,255.51 | 24.63 | 2.50 | 39,091.68 | 19.55 | 2.15 | 33,618.84 | 16.81 |
6-12
| 1.60 | 28,281.98 | 14.14 | 1.38 | 24,393.21 | 12.20 | 1.25 | 22,095.30 | 11.05 |
0-12 | 2.33 | 77,537.49 | 38.77 | 1.91 | 63,484.89 | 31.74 | 1.67 | 55,714.14 | 27.86 |
Table 3. Mean total organic carbon at two soil depths and at three dates as a percentage, pounds per acre, and tons per acre from three treatments, twice-over, seasonlong, and nongrazed, 2022 and 2023. | ||||||
---|---|---|---|---|---|---|
| 2022 | 2023 | ||||
Soil Depth inches |
OC% |
lbs/ac |
tons/ac |
OC% |
lbs/ac |
tons/ac |
Twice-over |
|
|
|
|
|
|
0-6 | 5.31 | 83,082.84 | 41.54 | 5.18 | 81,050.08 | 40.53 |
6-12 | 2.58 | 45,604.69 | 22.80 | 3.17 | 55,974.75 | 27.99 |
0-12 | 3.86 | 128,687.53 | 64.34 | 4.11 | 137,024.83 | 68.51 |
Seasonlong |
|
|
|
|
|
|
0-6 | 4.02 | 62,859.42 | 31.43 | 4.85 | 75,837.85 | 37.92 |
6-12 | 1.86 | 32,936.72 | 16.47 | 2.13 | 37,709.30 | 18.85 |
0-12 | 2.88 | 95,796.14 | 47.90 | 3.41 | 113,547.15 | 56.77 |
Nongrazing |
|
|
|
|
|
|
0-6 | 2.52 | 39,456.53 | 19.73 | 2.60 | 40,655.34 | 20.33 |
6-12 | 1.53 | 27,044.64 | 13.52 | 1.41 | 24,923.50 | 12.46 |
0-12 | 2.00 | 66,501.17 | 33.25 | 1.97 | 65,578.84 | 32.79 |
Literature Cited
Anonymous. 2022. The earths carbon pools. Kansas State Univ. 4p.
Anonymous. 2023. Carbon dioxide in Earth’s atmosphere. wikipedia.org. 25p.
Barker, W.T., and W.C. Whitman, 1988. Vegetation of the Northern Great Plains. Rangelands 10:266-272.
FAO and ITPS. 2015. Status of the world’s soil resources, Food and Agriculture Organization of the United
Nations and Intergovernmental Technical Panel on Soils. Rome, Italy. 605p.
FAO. 2017. Soil organic carbon: the hidden potential. Food and Agriculture Organization of the United Nations. Rome, Italy. 77p.
FAO. 2023. Global assessment of soil carbon in grasslands. Food and Agriculture Organization of the United
Nations. Paper No. 187. Rome, Italy. 52p.
Gibson, D.J. 2009. Grasses and grassland ecology. Oxford University Press Inc. New York, NY. 305p.
Keenan, T.F., and C.A. Williams. 2018. The terrestrial carbon sink. Annual Review of Environmental and Resources. 43:219-243.
Manske, L.L. 2013. Perpetually sustainable grazingland ecosystems. NDSU Dickinson Research Extension
Center. Summary Range Management Report DREC 13-3060. Dickinson, ND. 4p.
Manske, L.L. 2018. Restoring degraded grasslands. pp. 325-351. in A. Marshall and R.Collins (eds.). Improving grassland and pasture management in temperate agriculture. Burleigh Dodds Science Publishing, Cambridge, UK.
Manske, L.L. 2024. Range plant growth related to climatic factors of western North Dakota, 1982-2023. NDSU Dickinson Research Extension Center. Range Research Report DREC 24-1078m. Dickinson, ND. 22p.
Rosen, J. 2021. The science of climatic change explained: facts, evidence, and proof. The New York Times. nytimes.com. 20p.
Russelle, M.P. 1992. Nitrogen cycling in pastures and range. Journal of Production Agriculture 5:13-23.
Schimel, D.S. 1995. Terrestrial ecosystems and the carbon cycle. Global Change Biology 1(1):77-91.
Schimel, D.S., B.H. Braswell, and W.J. Parton. 1997. Equilibrations of the terrestrial water, nitrogen, and carbon cycles. Proceedings of the National Academy of Science, USA. 94:8280-8283.
Schimel, D., B.B. Stephens, and J.B. Fisher. 2015. Effects of increasing CO2 on the terrestrial carbon cycle. Proceedings of the National Academy of Science, USA. 112(2):436-441.
Shiflet, T.N. (ed.). 1994. Rangeland cover types. Society of Range Management. Denver, CO. 152p.
Working Group I. 2007. Historical overview of climate change science. The fourth assessment report on climate change. Cambridge University Press, Cambridge, United Kingdom, and New York, NY. 35p.
Proactive Preventive Pestiferous Grasshopper Habitat Management
Llewellyn L. Manske PhD
Scientist Of Rangeland Research
North Dakota State University
Dickinson Research Extension Center
Report DREC 24-4041
Grasshoppers are a natural component of native rangeland and domesticated grassland pastures of the Northern Plains. Grasshopper population numbers are precariously balanced between their high fecundity with the capacity to rapidly reproduce themselves several times over, and the enormous mortality rate inflicted by the numerous pathogens, parasites, and predators that are constant natural enemies. When grasshoppers are at low numbers, they can be supported by the resources of the grassland ecosystem, and grasshoppers are not a problem. However, when changes in environmental conditions cause decreases in mortality rates, increases in successful egg hatches, and/or increases in access to direct sunlight, pestiferous rangeland grasshopper populations can greatly increase to problematic outbreak levels that can consume and destroy immense quantities of the valuable available livestock forage (Manske 2014).
Control measures after the grasshopper assemblages have reached unacceptable outbreak numbers have moved past the effectiveness of preventative practices and require curative practices using expensive pesticides. Most insecticides are nonselective and are also lethal to nontarget beneficial or neutral insects. Predators that consume dosed insects are also detrimentally affected by the pesticide. All pesticides are toxic and have some level of propensity towards harmful risks to health of humans and the environment. To reduce the undesirable effects from chemical pesticides, it would be advantageous to restrict insecticide use to emergencies only, and primarily depend on use of proactive preventative management practices as the preferential alternative.
Proactive preventative pestiferous grasshopper habitat management is science based biologically effective natural pest control practices that interfere with the grasshopper life cycle by changing the habitat characteristics to be less favorable for the survival, growth, development, dispersal, and reproduction of pestiferous grasshoppers on grassland pastures of the Northern Plains. Proactive preventative management practices require long-range planning and detailed knowledge of grasshopper life cycle, including development of egg, nymph, and adult, thermoregulation, food requirements, and habitat characteristics, along with grassland ecosystem mechanisms and processes, and rhizosphere organism biogeochemical processes (Manske 2021).
Grasshopper Life Cycle
Grasshoppers in the Northern Plains have a one year life cycle with an active period that occurs, during most years, from early May to mid October. This active period is exactly the same 5.5 month period of active grass growth of native graminoids. Grasshoppers transition by simple metamorphosis through three life stages; egg, nymph, and adult. The majority of grasshoppers’ life cycle is egg-nymph-adult-egg. A few grasshopper species spend the winter period as an hibernating mid to late stage nymph. Their life cycle is nymph-adult-egg-nymph.
Grasshopper egg hatch usually occurs from early May to late July. Eggs of grasshopper species tend to hatch during the same periods each year. The seasonal hatch periods of different species have been categorized into five
hatching groups. The very early (VE) hatch occurs from late April to early May; the early (E) hatch occurs from mid to late May; the intermediate (I) hatch occurs from early to mid June; the late (L) hatch occurs from mid to late June; and the species that overwinter in the nymph stage have a very late (VL) hatch that occurs from mid to late July. The actual hatch time depends on the total amount of accumulated heat units received by the eggs (Cushing 1993, 1996; Pfadt 1994; Cushing et al. 1996).
Embryonic development begins immediately after egg deposition. The embryos receive nourishment from the yolk (Pfadt 1994). The rate of development depends on heat units measured in day-degrees DD. Day-degrees are accumulation of degrees each day that are above the threshold temperatures of 50° or 55° F (10° or 13° C). Grasshopper eggs require about 400 DD day-degrees of heat by fall in order for development to reach embryo stage 19, which is 50% developed, and begin diapause (Pfadt 1994). Diapause stops growth and metabolic activity to prevent completion of development and hatching during unsurvivable weather (Fisher et al. 1996a). Cold soil temperatures end diapause and the embryos enter into a dormant state until spring (Watts et al. 1989). During the next spring, the embryos resume development and require an additional 150 DD of heat to reach stage 27 (100%) and hatching (Pfadt 1994, Fisher et al. 1996a). The embryos of a single egg pod hatch together, wiggle to the soil surface and squirm out of an embryonic membrane, serosa, then they are able to stand upright, jump, and they are ready to start feeding (Pfadt 1994).
Most grasshopper species develop through five immature instar stages. Some large females develop through six instar stages. From hatching to adult stage usually requires 35 to 50 days, at a rate of 7 to 10 days per instar. With the fifth or last instar molt, the nymph becomes an adult, or imago. The new fledging adult has fully functional wings, however, it requires an additional 1 to 3 weeks to increase in weight and to complete maturation of reproductive organs (Pfadt 1994).
The male grasshoppers usually hatch a little ahead of the females. Males actively search for mature females. The courtship varies with species. Grasshoppers are able to identify other members of its species. Grasshoppers communicate through visual and audible signals (Pfadt 1994). If the female is enamored by the males courtship activities, they mate, if not, she ignores him or literally kicks him out.
Following mating, the females require a period for egg development. The gravid female deposits a clutch of eggs in a hole in the soil. The soil is tested for physical and chemical properties. The selected egg laying site is evaluated by soil texture, temperature, moisture level, acidity, salt content, size of bare area, and type of vegetation (Pfadt 1994). The female digs a hole in the soil about 0.5 to 1.5 inches (10 to 40 mm) deep. The female has three pairs of digging and egg laying structures. Egg pod depth varies, 7-12 mm is shallow, 13-24 mm is medium, and 25-38 mm is deep. The sides of the hole are lined with a secreted froth that becomes the egg pod (Davidowitz 2013). The clutch of eggs is deposited in the pod. The number of eggs per clutch varies from, 1 to 90. A female can deposit a clutch about every 2 or 3 days and produce 4 to 25 egg pods. One female typically produces a maximum of 100 to 200 eggs (Cushing 1193, 1996, Pfadt 1994, Cushing et al. 1996).
Thermoregulation
Grasshoppers are cold blooded (ectothermal). They are unable to regulate their body temperature metabolically. Their body temperature varies with the surrounding environment. In order to increase their body temperature, they absorb heat by basking in direct sunlight. In order to reduce their body temperature, they elevate their body above the soil on the shady side of vegetation and hyperventilate to increase the volume of air moving in and out of their tracheae causing evaporative cooling (Carruthers et al. 1992).
The rate of embryonic development in the egg depends on the quantity of heat units received from direct sunlight on the soil near the egg pod. The rate of nymphal development is determined by the nutritional quality of the food plants and by the amount of time the nymphs can raise their body temperatures to optimal levels through basking in unobstructed direct sunlight. The rates of adult growth and development, metabolism, activity levels, and the speed of escaping from predators depends on the proportion of each day they can maintain their preferred optimal body temperature by the exposure to direct rays from the sun plus the rays reflected from the bare ground (Parker 1982).
Grasshopper nymph and adult daily activities start shortly after dawn. During the night, their body temperature is the same as the environment. The grasshopper crawls on the ground to an open spot that receives unobstructed direct radiant rays from the sun. The common basking position is to turn its side perpendicular to the sun rays and lower the associated hindleg. They turn around and expose the opposite side and also expose their back. The incident solar radiation raises their internal body temperature above ambient levels. The preferred optimal body temperature is 95° to 104° F (35° to 40° C) (Parker 1982, Carruthers et al. 1992). The morning basking period usually lasts 1 to 2 hours. Maintenance of optimal body temperature requires a constant daily behavioral rhythm to move from shade to sun, and all activity movements are related to the solar position (Jech 1996).
Food Requirements
All grasshoppers are herbivorous. Those that eat grass are graminivores. Those that eat forbs are forbivorous. A few are mixed feeders and eat both grasses and forbs. About half of the nasty pestiferous grasshoppers are polyphagus and eat many different kinds of food plants including grasses, forbs, and agricultural crops. Most of the pestiferous grasshoppers prefer to feed by climbing on the food plant. Some grasshoppers prefer to feed on the food plant from the ground, while some grasshoppers can feed from the ground or on the food plant. All of the substances necessary for growth, reproduction, and maintaining life processes in grasshoppers must come from the food plants that they eat (Joern 1996a, b).
Grasshoppers require a diet that provides adequate protein, energy, water, minerals, and vitamins. Protein is the most limiting nutrient. Protein makes up 50% of the cuticle of the exoskeleton. They develop new exoskeletons for each instar stage and when they become adults. Muscle and organ tissue contain protein. Digestive enzymes and hemolymph (body fluid) require protein. Female ovarian growth and egg formation requires large amounts of the protein vitellogenin. Energy is obtained from simple sugars and soluble starches in plants. Grasshoppers are cold blooded (ectothermal) and can not regulate their body temperature metabolically, so they do not require energy at the levels needed by mammals. Liquid water has limited availability in arid and semiarid regions that do not have dew in the morning. Water, then, must come from the food plants. The amount of water in the leaves could influence preference for food plants. Green grass leaves usually have high water content at 60% to 80%. The exoskeleton of grasshoppers is efficient at conservation of water. Macromineral, micromineral, and vitamin requirements for grasshoppers is not known but it most likely would be similar to other living creatures (Joern 1996a, b).
Most grasshopper species are extremely discriminating when selecting food plants. The grasshopper approaches a potential food plant, lowers their antennae to the leaf surface and then drum or tap on the leaf with their maxillary and labial palpi. These sensory organs can separate the properties of plant chemicals into attractants or repellents. The grasshopper rejects the unfavorable plants and chooses the favorable host plants. The manible cuts the leaf into bit size pieces and starts mechanical digestion. The salivary glands secrete chemical enzymes that digest carbohydrates. The pharynx and esophagus are located in the buccal cavity and lead to the crop that holds food and starts protein digestion. The gizzard has hard tooth-like features that breakup food. The stomach mixes chemical enzymes with the food to break it down. The gastric caecum surrounds the stomach, secretes digestive enzymes, protease, lipase, amylase, invertase, and several others, and absorbs amino acids. The peritrophic membrane continuously produces protein/chitin complex. The ileum section of the intestine continues food digestion and absorbs soluble food matter and water. The malpighian tubules excrete uric acid, urea, and amino acids into the rectum section of the intestine where dry pellets are formed from the food residue and waste products that are then disposed of through the anus (Joern 1996a, b). Feeding continues long enough to fill the grasshoppers crop and the feeding sessions stop until the crop is empty. While the crop empties, the grasshopper walks around aimlessly; this behavior is called pottering. The grasshopper repeat the cycle of feeding sessions and pottering (Jech 1996).
Active growth of the graminoids in the Northern Plains provides adequate nutrient quality during the same active growth period of the grasshoppers. Native cool season grass lead tillers start early leaf greenup as vegetative carryover tillers in mid April with crude protein levels above 16% by mid May. By the 3.5 new leaf stage near early June, crude protein levels are still above 15%. They reach the flower stage before 21 June, when crude protein begins to decrease below 15%. The crude protein levels remain above 9.6% until mid July. As the lead tillers mature, the fiber content increases, and percent crude protein, water, and digestibility decreases. During late July, crude protein levels drop below 8.0% and below 6.5% in late August. Crude protein levels of cool season secondary vegetative tillers increase above 9.6% during July and August to 13.2% in early September, decrease during September, and drop below 9.6% in early to mid October (Whitman et al. 1951, Sedivec 1999, Manske 2008a).
Native warm season grass lead tillers start early leaf greenup as vegetative carryover tillers in mid May with crude protein levels above 15% by early June. Around mid June, the 3.5 new leaf stage is reached with above 13% crude protein. The flower stage is reached after 21 June with 12.2% crude protein. While the seeds develop, the crude protein levels remain above 9.6% until late July. As the lead tillers mature, the fiber content increases and percent crude protein, water, and digestibility decrease. During mid August, crude protein levels drop below 7.0%, and below 6.0% by early September. Crude protein levels of warm season secondary vegetative tillers increase above 9.0% during August to 10.0% in early September, decrease during September, and drop below 9.6% in late September (Whitman et al. 1951, Sedivec 1999, Manske 2008a).
Upland sedges grow very early with crude protein levels above 9.6% from late April to early May until mid July, when plants increase in senescence and crude protein drop below 7.8% in early August but do not fall below 6.2% for the remainder of the growing season (Whitman et al. 1951, Manske 2008b).
Phosphorus levels drop below 0.18% when plants reach the mature seed stage, in late July for cool season grasses, in late August for warm season grasses, and in mid May for upland sedges (Whitman et al. 1951, Goetz 1963, Sedivec 1999, Manske 2008a, b).
Habitat Characteristics
Most grasshopper species are able to successfully occupy a wide variety of prairie types throughout North America (Onsager 1998). The pestiferous rangeland grasshopper species of the Northern Plains have extensive distribution across the short grass prairie, the mixed grass prairie, the tall grass prairie, the sandhills prairie, and the bunch grass prairie.
The grassland conditions in which a grasshopper species performs best and develops the highest population densities is considered to be the preferred habitat. Many of the pestiferous rangeland grasshoppers prefer habitat with short grass areas mixed amongst many open bareground patches. The short grass areas can be located on dry upland sites dominated by blue grama and upland sedges or sites with mid and tall grass species heavily grazed to a short stature. Most of the pestiferous rangeland grasshoppers prefer habitat with areas that have a mixture of mid and short grasses interspersed among numerous bareground patches. A few of the pestiferous rangeland grasshoppers prefer habitat with deteriorated mid and tall vegetation on moist meadows, invaded by weeds, and containing many open bareground patches. All of these grasshopper preferred grassland habitat conditions are abundantly present within all of the Northern Plains prairie types. The universally important habitat characteristic is the numerous open bareground patches (Mulkern et al. 1969, Pfadt 1994, Cushing 1996, Manske 2014). Pestiferous grasshoppers require bareground patches for egg pod deposition sites and for basking in unobstructed sunlight to raise their body temperatures to optimal levels.
The depth of deposition of the egg pods is an intrinsic characteristic of a grasshopper species. Few grasshopper species deposit their egg pods at shallow depths. Many grasshoppers deposit their egg pods at medium depths. Most grasshoppers deposit their egg pods at deep depths. It would seem reasonable to expect that the egg pods deposited at shallow depths should have the advantage of location and accumulate degree days of heat more rapidly than the egg pods deposited at medium and deep depths, and that the shallow deposited egg pods should have more rapid embryonic development and earlier hatch. All of the grasshopper species that deposit their egg pods at shallow depths hatch with the early group during mid to late May. However, some of the grasshopper species that deposit their egg pods at medium depths also hatch with the early group. Remarkably, a few of the grasshopper species that deposit their egg pods at medium and deep depths hatch earlier with the very early group during late April to early May. This insightful information indicates that the depth of egg deposition is not the most important factor that determines the rate of embryonic development and when the eggs will hatch (Cushing 1993, 1996, Pfadt 1995, Manske 2014).
Most of the pestiferous rangeland grasshoppers of the Northern Plains deposit their egg pods at medium or deep depths. The deeper depths provide greater protection from cold air temperatures during the winter. The greater protection from cold air temperatures provided by deeper egg pod depths must give northern grasshoppers greater advantages and increased survival rates than rapid accumulation of degree days of heat provided by shallow egg pod depths.
A little more than half of the pestiferous grasshoppers hatch before early June; with a few that hatch very early during late April to early May, and many that hatch early during mid to late May. A little less than half of the pestiferous grasshoppers hatch after early June, with the intermediate and late groups during early to late June. Only a very few pestiferous grasshoppers overwinter in a late nymphal stage, deposit egg pods at a medium depth, and hatch very late during mid to late July in the same growing season that they were laid (Cushing 1993, 1996, Pfadt 1994, Manske 2014).
All of the pestiferous rangeland grasshoppers are geophilous (earth loving) conducting most of their daily activities of courting, mating, pottering, and resting on the ground, and also doing their basking on bareground areas to accumulate thermal heat from unobstructed sunlight and also take advantage of the additional rays reflected off the nearby bare soil for the purpose of increasing their body temperatures to optimal levels. A few pestiferous grasshoppers are also phytophilous (plant loving) conducting some of their daily activities in vegetation, and also sometimes climbing unto vegetation to heights that receive direct sunlight as another primary basking site. However, basking in vegetation is less effective than basking on bareground (Pfadt 1994).
Grasshoppers as a problem
Hexapod insects (six-legged) formed during the Silurian period around 410 million years ago (mya). Shorthorned grasshoppers (Caelifera) split from the longhorned crickets (Ensifera) near the Permian-Triassic boundary around 251 mya (Anissimov 2013). Flowering plants (Angiosperms) started to evolve during the Early Cretaceous around 120 mya. Ancestral grass fossil silica bodies (phytoliths) have been described from the Albian age, late Early Cretaceous, 113 mya (Prasad et al. 2005, 2011, Poinar 2004, 2011, Wu, You, and Li 2018). During the mid to late Cretaceous, numerous new herbivorous dinosaur species ate grass plants (Manske 2022). The grasshopper subfamilies of Gomphocerinae, Melanoplinae, and Oedipodinae developed between 90 and 50 mya during the Late Cretaceous and Early Paleogene. Near the end of the Eocene epoch around 34 mya, the global climate cooled resulting in reduced forest ecosystems and increased open grasslands (Chintanan-Marquier et al. 2011, Gomez et al. 2012, Anissimor 2013): Large mammalian graminivores grazed these expansive grasslands during the Oligocene, Miocene, until the end of the Pleistocene, 34 to 0.012 mya (Hibbard 1958, Stebbins 1981, Bell et al. 2004, Dyke 2005, Prothero 2006, Osborne 2008, Semprebon et al. 2019).
Grasshoppers are a natural and integral component of grassland ecosystems. Usually grasshopper populations remain at densities that can be supported by the ecosystem. Unfortunately, occasionally the grasshopper populations increase to outbreak levels. Weather variables have sometimes been correlated with grasshopper population dynamics, however, weather variables are not the direct cause but do effect some factors that can change grasshopper population numbers (Fisher et al. 1996b, Belovsky 2000). The mechanisms that drive grasshopper population numbers are changes in plant production and nutritional quality, changes in grasshopper growth and development rates, and changes in predation, parasitism, and pathogen infection rates. Grasshopper growth and development rates are determined by the nutritional quality and quantity of the food plants and by accessibility to thermal heat units from the sun. Grasshopper population numbers are affected by the mortality rate caused by natural enemies, and their quantities are indirectly determined by environmental conditions (Onsager 2000, Manske 2014).
Grasshopper populations in the semiarid, subhumid, and humid regions of the Northern Plains are not limited by the quantity of available nutritious food plants. Northern Plains grasshoppers are primarily limited by the accessibility to thermal heat units from the sun because the relatively tall vegetation canopy can cover and shade the bareground areas used as basking sites. Grasshopper populations in the Northern Plains are usually suppressed during growing seasons with normal and above normal precipitation that produces normal and above normal grass herbage biomass. Generally, Northern Plains grasshopper populations increase when vegetation canopy height decrease. On native grasslands, grass canopy height decreases during low precipitation conditions or when livestock are permitted to graze heavy. On domesticated grasslands, grass canopy height decreases when the pasture receives double use during one growing season, such as spring grazing and summer haying, or spring and fall grazing. Any of the factors that cause reductions in vegetation height results in greater accessibility to thermal heat units from the sun permitting grasshopper populations to increase. The greater the size of bareground area, the greater the accessibility to thermal heat units from the sun, the higher the grasshopper population infestation will be (Onsager 1987, 1996, 1998, 2000; Manske 2014).
Vegetation canopy reduction or removal increases solar radiation and day degree heat units at the soil surface and increases air flow over the ground, which increases both the soil and air temperatures and decreases relative humidity for grasshoppers. Increased solar radiation to the soil increases day degree heat units that accelerate embryonic development in the egg that results in earlier hatch. Vegetation canopy reduction improves basking sites that hasten body warm up of nymphs and adults that shorten instar stages and maturation time. Greater solar radiation, higher soil and air temperatures, and lower humidity in grasshopper microhabitat has debilitating effects on pathogens, resulting in lower mortality rates, which increases the number of nymphs reaching adult stage, and increasing the longevity of adults that promotes greater egg production (Onsager 1987, 1996, 1998, 2000; Manske 1993, 1994a, 2014).
Reduction of the number and size of the bareground areas in pestiferous rangeland grasshopper habitat reduces the number of days and hours per day that grasshopper thermoregulation of body temperature reaches or stays at the optimal level. Metabolic rates and processes are reduced, digestion rates are diminished, food intake is decreased, and growth and development are slowed down resulting in higher mortality of juveniles, fewer adults fledge, maturation of adults is lengthened, the time period for egg production is shortened, the quantity of viable eggs deposited is reduced, causing decreased grasshopper densities and lower grasshopper populations (Onsager 1998, 2000, Manske 2014).
Effects of Grazing Systems on Grasshopper Populations
Grazing systems cause changes to vegetation structure. It should be possible for a grazing system to effectively cause changes to grasshopper habitat and control population abundance. A collaborative project with a range scientist (Dr. Manske) and an entomologist (Dr. Onsager) was conducted for six years in western North Dakota to document that a grazing system could change the grasshopper habitat sufficiently enough to suppress grasshopper population outbreaks.
The traditional seasonlong grazing system on native rangeland was grazed from 1 June to 14 October at 2.58 ac/AUM producing a mean monthly residual standing vegetation biomass of 1359.07 lbs/ac. The aboveground herbage biomass consisted of 11.6% standing dead and 88.4% live herbage with 56.7% native grass (39.7% cool season and 16.8% warm season), 14.2% upland sedge, and 17.6% forbs (table 1).
The twice-over rotation grazing system on native rangeland was grazed from 1 June to 14 October at 2.26 ac/AUM producing a mean monthly residual standing vegetation biomass of 1597.31 lbs/ac. The aboveground herbage biomass consisted of 15.5% standing dead and 84.5% live herbage with 63.3% native grass (45.0% cool season and 18.3% warm season), 10.6% upland sedge, and 10.7% forbs. The twice-over grazing system produced 17.5% greater total standing residual herbage biomass than the traditional seasonlong grazing system (table 2).
Basal cover of live vegetation on the traditional seaosnlong grazing system was 23.7% and was 32.1% on the twice-over rotation grazing system. Live vegetation basal cover was 35.6% greater on the twice-over grazing system (tables 3 and 4).
Bare soil measured by the ten-point frame method was 7.63% on the traditional seasonlong grazing system and was 6.35% on the twice-over grazing system. Bare soil not covered by live plants or litter was 20.2% greater on the seasonlong grazing system (tables 3 and 4).
The traditional seasonlong grazing system had shorter height structure with relative cover of 46.3% short grass, and had basal cover composition with 69.2% greater warm season grass and 45.4% greater upland sedge than those on the twice-over grazing system (table 5).
The twice-over rotation grazing system had taller height structure with relative cover of 53.8% mid height cool season grass, and had basal cover composition with 101.1% greater cool season grass than those on the traditional seasonlong grazing system (table 5).
The residual vegetation structure on the traditional seasonlong grazing system was primarily short grass and upland sedge which was favorable pestiferous rangeland grasshopper habitat. The residual vegetation structure on the twice-over rotation grazing system was primarily mid height cool season grasses which was unfavorable pestiferous rangeland grasshopper habitat.
Grasshopper population density data was collected one or two times per week in each study pasture from a set of 40 aluminum wire rings, each 0.1m2, total area of 4 m2, affixed to the ground in a 4 X 10 array with 8 meters between rings as described by Onsager and Henry 1977. Field data was converted into grasshopper days (GD) per m2, similar to the concept of animal unit months (AUM) (Onsager 2000). Mean cumulative grasshopper days (GD) per m2 were significantly greater on the seasonlong grazing system than on the twice-over grazing system (Onsager 2000). The seasonlong grazing system had an annual mean of 358 GD for the 9 pestiferous rangeland grasshoppers and had an annual mean of 748 GD for the total of all grasshopper species (table 6). The twice-over grazing system had an annual mean of 122 GD for the 9 pestiferous rangeland grasshoppers and had an annual mean of 229 GD for the total of all grasshopper species (table 6).
The Migratory grasshopper had elevated annual mean grasshopper days per m2 on both grazing systems with 87 GD on the seasonlong grazing system and 78 GD on the twice-over grazing system (table 6). Population abundance of four pestiferous grasshopper species, Gladston, Obscure, Dusky, and Little Spurthroated grasshoppers increased significantly to annual means of 88 GD, 71 GD, 57 GD, and 26 GD, respectively, on the seasonlong grazing system, while remaining at low annual means of 3 GD, 6 GD, 10 GD, and 5 GD, respectively, on the twice-over grazing system (table 6). No grasshopper species had population abundance at significantly greater grasshopper days on the twice-over grazing system (table 6). Four pestiferous grasshopper species, Largeheaded, Redlegged, Kiowa, and Whitewhiskered grasshoppers had low abundance on both seasonlong and the twice-over grazing systems (table 6).
Grasshopper populations on the seasonlong grazing system had moderate densities between 3.8/yd2 and 6.9/yd2 with moderate monthly forage use between 38.6 lbs/ac and 80.8 lbs/ac during 1993 to 1995 (table 7). Grasshopper populations on the twice-over grazing system had low densities between 1.2/yd2 and 2.8/yd2 with low monthly forage use between 14.2 lbs/ac and 36.2 lbs/ac during 1993 to 1995 (table 7). Forage use determined by Hewitt and Onsager 1982a method.
Grasshopper population assemblage increased to outbreak levels in 1997 and 1998 on the seasonlong grazing system (figure 1). Grasshopper densities on the seasonlong grazing system increased greatly to 15.4/yd2 in 1997 with high monthly forage use of 186.5 lbs/ac and the densities increased further to 22.4/yd2 in 1998 with large monthly forage use of 307.7 lbs/ac (table 7).
This huge grasshopper population outbreak did not occur on the twice-over grazing system (figure 1). Grasshopper densities on the twice-over grazing system remained low at 1.8/yd2 in 1997 with low monthly forage use of 21.2 lbs/ac. The densities increased slightly to 4.7/yd2 in 1998 with monthly forage use raising to 47.7 lbs/ac (table 7).
The residual vegetation structure on the seasonlong grazing system was comprised primarily of short stature warm season grass and upland sedge (table 5) which was the major factor that permitted the grasshopper population assemblages to greatly increase above outbreak levels. The outbreak assemblage consisted of three major pestiferous species, Gladston, Obscure, and Dusky (table 6). All three species hatch with intermediate or late groups after early June which reduces the length of development time to hard frost by greater than a month compared to the hatch time of the very early or early groups that occurs from late April to late May.
In order for grasshopper species that hatch after early June to survive in northern habitats, nymphal development, adult growth and maturation, mating, egg development, and egg deposition needs to progress at near physiological capabilities. Sustentation of these high rates of growth and development depend on the body temperature to be at optimum during most of the daylight hours. Maintaining body temperature at optimum requires readily accessible direct sunlight. In order for late hatching grasshopper populations to increase above outbreak quantities, a huge proportion of the nymphal population must develop to the adult stage at near potential rates and successfully deposit large numbers of eggs during a minimum of two consecutive years.
The taller residual vegetation structure on the twice-over grazing system was comprised primarily of mid height cool season grass (table 5) that restricted grasshopper access to direct sunlight decreasing the length of time that body temperature could be maintained at optimum levels causing reduction in the rates of growth and development that delayed adult maturation and egg development impeding egg deposition and thus preventing the population assemblage from increasing to outbreak numbers,
The vegetation structure on the twice-over grazing system successfully prevented population increases of all the grasshopper species that hatched after early June with the intermediate and late groups, the Dusky, Redlegged, Kiowa, Gladston, Obscure, and Largeheaded grasshoppers, and also prevented population increase of the grasshopper species hatching before June with the early group that preferred to bask on bareground, the Little Spurthroated and Whitewhiskered grasshoppers. The vegetation structure on the twice-over grazing system was not fully successful at preventing population increases of the grasshopper species hatching with the early group that were capable of basking both on bareground and on vegetation, the Migratory grasshopper.
Onsager (2000) determined that nymphal development of the 3rd instar to the 5th instar of the Migratory grasshoppers was significantly slower and required 2.6 days longer for development per instar on the twice-over grazing system with a mean of 11.5 days per instar than that on the seasonlong grazing system with a mean of 8.9 days per instar resulting in a delay of 7.8 days for the appearance of adults on the twice-over grazing system. This delay in nymphal development had a major impact on the proportion of the nymphal population that became adults. The population density counts conducted by Onsager (2000) revealed that the twice-over grazing system with a mean density of 0.60 adults/m2 (0.5/yd2) produced fewer adult grasshoppers than that produced on the seasonlong grazing system with a mean density of 2.05 adults/m2 (1.71/yd2). Onsager (2000) gave the most plausible explanation that would cause this measured delay in instar development. The vegetation structure on the twice-over grazing system interfered with the amount of direct sunlight reaching the grasshopper microhabitat causing the ambient air temperature to become cooler preventing efficient thermoregulation of nymphal body temperature. In addition, Onsager (2000) showed that the slightly lower biological survival rate for nymphs on the twice-over grazing system over the longer delayed development time for three instar stages could result in the great difference in the measured densities of adult grasshoppers with 70.7% fewer adult grasshoppers per square meter on the twice-over grazing system than on the seasonlong grazing system. Greatly reduced densities of adult grasshoppers would deposit lower quantities of viable eggs that season and produce fewer hatchlings the next growing season.
The twice-over rotation grazing system effectively changes the characteristics of grasshopper habitat to be unfavorable for growth and development of large assemblages of pestiferous grasshoppers and is an effective proactive preventive management practice that can suppress grasshopper outbreaks on the grasslands of the Northern Plains.
Table 1. Mean monthly residual herbage biomass (lbs/ac) on the silty ecological site of the Traditional Seasonlong grazing system. | ||||||
---|---|---|---|---|---|---|
Plant Biotype |
Jun |
Jul |
Aug |
Sep |
Oct | Mean Monthly |
cool season | 483.96 | 606.10 | 515.39 | 548.70 | 542.12 | 539.25 |
warm season | 157.64 | 244.45 | 287.08 | 222.68 | 241.69 | 230.71 |
sedge | 226.16 | 237.83 | 222.50 | 151.45 | 126.55 | 192.90 |
forbs | 218.24 | 293.73 | 253.01 | 212.18 | 216.13 | 238.66 |
total live | 1086.00 | 1382.11 | 1277.98 | 1135.01 | 1126.49 | 1201.52 |
st. dead | 131.66 | 152.88 | 156.66 | 184.01 | 162.60 | 157.55 |
total biomass | 1217.60 | 1534.99 | 1434.64 | 1319.02 | 1289.09 | 1359.07 |
Table 2. Mean monthly residual herbage biomass (lbs/ac) on the silty ecological site of the Twice-over grazing system. | ||||||
---|---|---|---|---|---|---|
Plant Biotype |
Jun |
Jul |
Aug |
Sep |
Oct | Mean Monthly |
cool season | 637.66 | 760.51 | 670.20 | 826.89 | 698.80 | 718.81 |
warm season | 217.06 | 304.43 | 333.21 | 300.86 | 302.53 | 291.62 |
sedge | 199.29 | 204.99 | 175.74 | 127.28 | 137.21 | 168.90 |
forbs | 146.55 | 193.27 | 187.79 | 164.72 | 159.88 | 170.44 |
total live | 1200.56 | 1463.20 | 1366.94 | 1419.75 | 1298.42 | 1349.77 |
st. dead | 206.79 | 195.98 | 334.48 | 238.79 | 261.65 | 247.54 |
total biomass | 1407.35 | 1659.18 | 1701.42 | 1658.54 | 1560.07 | 1597.31 |
Table 3. Plant basal cover (%) and composition (%) on the silty ecological site of the Traditional Seasonlong grazing system. | ||
---|---|---|
Plant Biotype | Basal Cover % | Composition % |
cool season | 5.85 | 24.70 |
warm season | 8.76 | 36.99 |
sedge | 5.95 | 25.13 |
forbs | 3.12 | 13.18 |
total live | 23.68 |
|
bare soil | 7.63 |
|
litter | 68.69 |
|
Table 4. Plant basal cover (%) and composition (%) on the silty ecological site of the Twice-over grazing system. | ||
---|---|---|
Plant Biotype | Basal Cover % | Composition % |
cool season | 15.95 | 49.67 |
warm season | 7.02 | 21.86 |
sedge | 5.55 | 17.28 |
forbs | 3.59 | 11.18 |
total live | 32.11 |
|
bare soil | 6.35 |
|
litter | 61.54 |
|
Table 5. Grass community height structure based on mean % relative cover of mid and short grasses. | ||
---|---|---|
Twice-over System % Relative Cover | Seasonlong System % Relative Cover | |
Mid Grasses | 53.76 | 42.90 |
western wheatgrass | 19.41 | 28.40 |
needle and thread | 3.68 | 1.29 |
green needlegrass
| 30.67 | 13.21 |
Short Grasses
| 28.20 | 46.31 |
prairie junegrass | 1.21 | 2.26 |
blue grama | 21.93 | 36.91 |
upland sedge | 5.07 | 7.15 |
Table 6. Mean dry weight (mg), forage use (lbs/ac/mo at 1/yd2), and mean cumulative grasshopper days (GD) per m2 on the twice-over and the seasonlong grazing systems. | ||||
---|---|---|---|---|
|
| Mean Cumulative Grasshopper Days | ||
Pestiferous Rangeland Grasshoppers | Mean Dry Weight mg | Forage Use at one/yd2 lbs/ac/mo | Twice-over Grazing GD/m2 | Seasonlong Grazing GD/m2 |
Gladston | 130.5 | 21.78 | 3 | 88* |
Migratory
|
131.5
|
21.29 |
78 |
87 |
Obscure
| 34.5 | 5.70 | 6 | 71* |
Dusky
| 92.5 | 15.95 | 10 | 57* |
Little Spurthroated | 58.0 | 9.59 | 5 | 26* |
Largeheaded | 100.0 | 16.79 | 6 | 16 |
Redlegged | 106.5 | 18.39 | 9 | 5 |
Kiowa | 62.0 | 10.33 | 3 | 6 |
Whitewhiskered | 60.0 | 7.60 | 2 | 2 |
Total GD/m2 for 9 pestiferous grasshoppers. | 122 | 358 | ||
Total GD/m2 for other 21 nonpestiferous grasshoppers. | 107 | 390 | ||
Total GD/m2 for all grasshoppers. | 229 | 748* |
Dry weight data from Pfadt 1994.
Forage use at one/yd2 data from Manske 2014.
Methods follow Hewitt and Onsager 1982a.
Grasshopper Days (GD) per m2 data from Onsager 2000.
Mean value GD is significantly greater than its complement*.
Table 7. Grasshopper density (per m2 and per yd2) and forage use per month (kg/ha and lbs/ac) on twice-over and seasonlong grazing systems. | ||||||
---|---|---|---|---|---|---|
Grazing Management System |
1993 |
1994 |
1995 |
1996 |
1997 |
1998 |
Twice-over |
|
|
|
|
|
|
Density |
|
|
|
|
|
|
#/m2 | 2.6 | 1.4 | 3.3 |
| 2.1 | 5.6 |
#/yd2 | 2.2 | 1.2 | 2.8 | 1.8 | 4.7 | |
Forage use |
|
|
|
|
|
|
kg/ha | 26.9 | 15.9 | 40.5 |
| 23.7 | 53.4 |
lbs/ac | 24.0 | 14.2 | 36.2 |
| 21.2 | 47.7 |
Seasonlong |
|
|
|
|
|
|
Density |
|
|
|
|
|
|
#/m2 | 8.3 | 4.6 | 6.2 |
| 18.4 | 26.8 |
#/yd2 | 6.9 | 3.8 | 5.2 |
| 15.4 | 22.4 |
Forage use |
|
|
|
|
|
|
kg/ha | 90.5 | 43.2 | 70.4 |
| 208.8 | 344.6 |
lbs/ac | 80.8 | 38.6 | 62.9 |
| 186.5 | 307.7 |
Density data from Onsager 2000.
Forage use data from Manske 2014.
Methods follow Hewitt and Onsager 1982a.
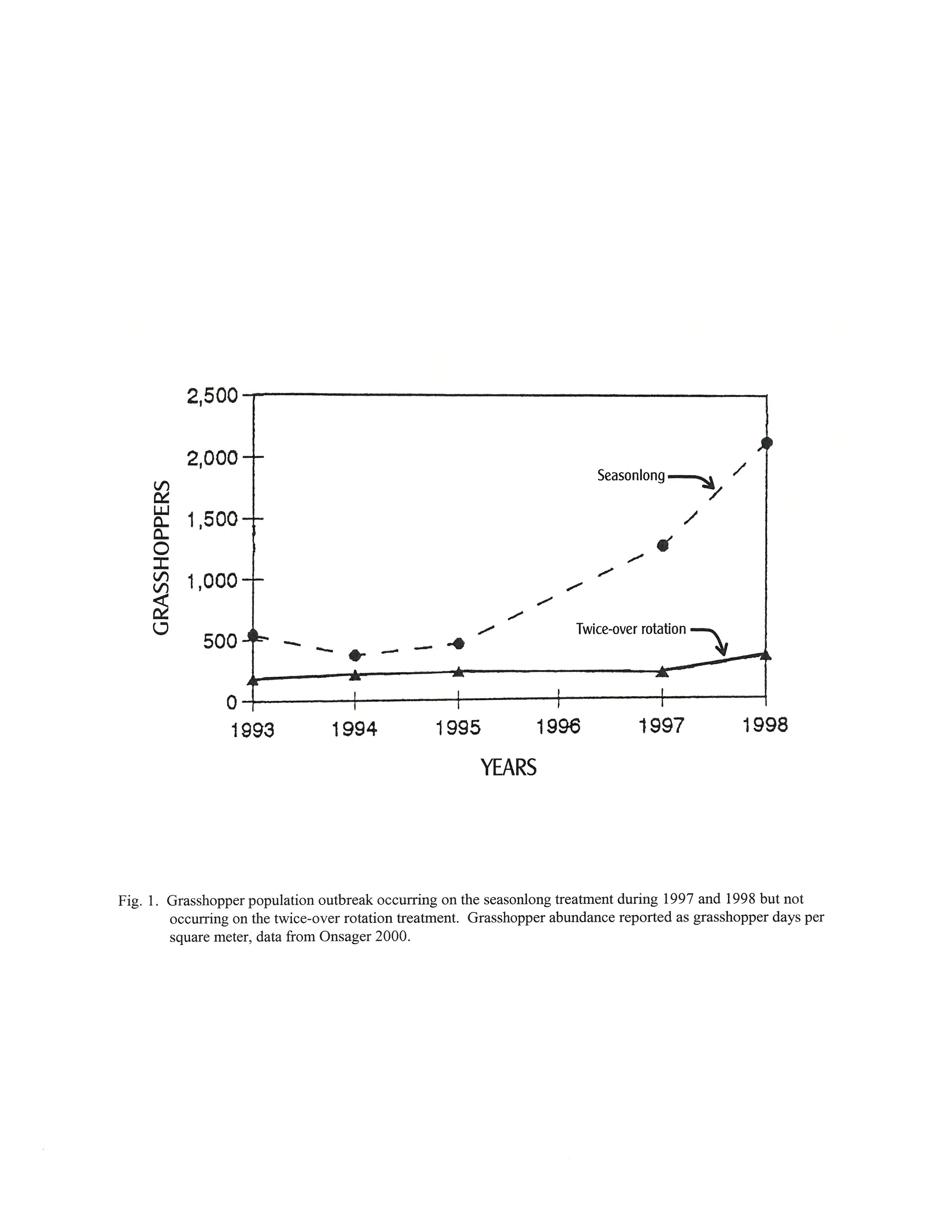
Grassland Ecosystem Mechanisms are Complex
Grassland ecosystems are composed of biotic and abiotic components. The indispensable biotic components are grass vegetation, soil rhizosphere organisms, and grazing graminivores which have biological and physiological requirements that must be met. The abiotic components consists of radiant energy from sunlight, climatic factors of precipitation and temperature, major essential elements of carbon, hydrogen, nitrogen, and oxygen, minor essential elements of macro-and-micro-nutrients, and environmental conditions including drought and fire.
Grass Tiller Growth and Development
The dominant type of reproduction in perennial grasses is vegetative tiller growth from axillary buds (Belsky 1992, Chapman and Peat 1992, Briske and Richards 1995, Chapman 1996, Manske 1999) not sexual reproduction and the development of seedlings. The frequency of true seedlings is extremely low, and establishment of an independent seedling in functioning grasslands is difficult and occurs only during years with favorable moisture and temperature conditions (Wilson and Briske 1979, Briske and Richards 1995), in areas of reduced competition from vegetative tillers.
Grass plants use the major and minor essential elements in the inorganic form to synthesize vital organic components of carbohydrates, proteins, and nucleotides for structural growth. Grass tillers consist of shoots and roots. The shoot is the stem and leaves comprised of repeated phytomers (Beard 1973, Dahl 1995). A phytomer consists of a leaf, with a blade and a sheath separated by a collar, a node, an internode, and an axillary bud (Hyder 1974, Dahl and Hyder 1977). Each tiller shoot produces 6 to 8 phytomers per growing season (Langer 1972, Dahl 1995). The crown of a grass tiller is the lower portion of a shoot and has two or more nodes (Dahl 1995). Fibrous roots grow from crown nodes that are located below ground. The internodes of the crown nodes associated with roots, crown tillers, and rhizome tillers do not elongate (Dahl 1995).
Longevity of grass tillers extends two grazing seasons (Langer 1956, Butler and Briske 1988, Manske 2009, 2014a). Phytomers develop from leaf primordia that form on alternating sides of the apical meristem (Evans and Grover 1940, Langer 1972, Beard 1973, Dahl 1995). Almost all of the phytomer cells are produced in the apical meristem while the leaf primordia is a minute bud (Langer 1972). The oldest cells of a leaf are at the tip, and the youngest cells are at the base (Langer 1972, Dahl 1995). Several leaf primordia are at various stages of development at any one time. The oldest leaf is outermost, while younger leaves grow up through existing leaf sheaths (Rechenthin 1956, Beard 1973). Growth of a leaf results through cell enlargement and elongation (Esau 1960, Dahl 1995). A few new cells are produced by intercalary meristem located at the base of the blade, the base of the sheath, and the base of the internode (Esau 1960). Cell expansion occurs in the region protected by the sheaths of older leaves. When the cells emerge and exposed to light, expansion ceases and photosynthesis and transpiration begin (Langer 1972). Once a leaf blade is fully expanded no further growth of that blade is possible (Dahl 1995).
Individual leaves of grass tillers are relatively short lived. Young middle-aged leaves are in their prime when the rate of apparent photosynthesis is maximum and the leaves begin exporting assimilates to other parts (Langer 1972). At this point, the leaf has its greatest dry weight. Leaf senescence, or aging, begins shortly after middle age. Senescence begins at the tip, the oldest part of the leaf, and spreads downward. As senescence progresses, apparent photosynthesis and movement of assimilates from the leaf to the other parts of the plant decrease (Langer 1972). The rate of senescence occurs at about the same rate as leaf appearance but is influenced by environmental conditions. Dryer soils increase rate of leaf senescence. During senescence, cell constituents are mobilized and redistributed to other parts of the plant (Beard 1973). This process causes weight of the leaf to decrease (Leopold and Kriedemann 1975). The percentage of dryness in a leaf blade is an indication of the degree of senescence.
Grass tillers remain vegetative during the first growing season, over winter, and resume growth as a lead tiller during the second subsequent growing season (Briske and Richards 1995) and generally does not exceed two growing seasons (Langer 1956, Butler and Briske 1988). Production of new leaf primordia continues until the status of the apical meristem changes from vegetative to reproductive (Dahl 1995, Briske and Richards 1995). Initiation of the reproductive growth stages is triggered during the second growing season by photoperiod (Roberts 1939, Dahl 1995) but can be slightly modified by temperature and precipitation (McMillan 1957, Dahl and Hyder 1977). Florally induced lead tillers develop sexual reproductive structures, the apical meristem ceases the production of leaf primordia and begins production of flower primordia when the lead tiller is between the third new leaf stage and the three and a half new leaf stage (Frank 1996, Frank et al. 1997). Previously formed leaf buds continue to grow and develop (Esau 1960, Langer 1972) until the flower stalk elongates (Dahl 1995). The flower bud primordia develop into the inflorescence with the apical dome becoming the terminal spikelet (Langer 1972). The life cycle of a lead grass tiller terminates during the end of the second growing season because production of additional leaves is no longer possible (Briske and Richards 1995).
Grass Plant Mechanisms
The previous concepts, before 2005, on how the evolutionary development of ancestral grasses occurred has been completely changed with the recent discovery of grass microfossils from the Cretaceous as old as 113 Ma (Manske 2022). This doubles the known age of grasses, which was 56 Ma from the Cenozoic. The much longer developmental timeline drastically changes the conditions of grass evolution. The early ancestral grasses developed in the closed canopy of gymnosperm forests that had a relatively warm wet habitat with no water deficiencies nor cold temperatures. The ancestral grasses had greatly reduced flower structures and were wind pollinated. They had a common trait that prevented cellular dehydration, but they could not survive very long in direct sunlight, or low temperatures, and they could not fully repair physical damage to leaves, stems, or roots. By the mid Cretaceous, the rapid angiosperm radiation was replacing the gymnosperms. Dense angiosperm trees grew along rivers, but open habitats of angiosperm shrubs and herbs were greatly increasing. In order for grasses to progress, they had to develop several complex survival mechanisms.
Mechanisms for Adaptation to Dry Open Habitats
With open habitats increasing, the ancestral grasses of the PACMAD clade and the Pooideae subfamily from the BOP clade shifted from the relatively wet environments of closed habitats with no water stress to drier open habitats with variable degrees of water stress. This huge shift in habitat conditions required the development of adaptive mechanisms to dry open habitats. These grasses developed deep branching fibrous roots with symbiotic arbuscular mycorrhizal fungi to absorb water and nutrients from the soil. They had to develop mechanisms to control the rate of water loss in order to prevent cellular dehydration but they had to have enough flexible responsiveness to be able to regulate and control gas exchange. These grasses also developed an elaborate vascular tissue system, complex leaves with water proof cuticles, and complex stomata with dumbbell shaped guard cells and lateral subsidiary cells. The grasses developed bulliform cells to roll or fold the leaves to reduce water loss. These improved structures provided fast action response to environmental changes in water balance resulting in improved water use efficiency permitting grasses to live in open habitats, with water deficient environments and still maintain significant biomass production. These grasses also developed mechanisms and phytohormones to repair and recover from tissue damage caused during water deficient conditions (Wang and Chen 2020).
Grazing Defense Mechanisms
Coincidental with the rapid diversification and great radiation of angiosperms came a huge improvement in the quantity and quality of available forage during the mid Cretaceous which resulted in an extensive explosion of new herbivorous dinosaurs (Manske 2022). Grasses progressively became a large portion of the dinosaurs diet. Within a short period, the intensified grazing pressure on grass ancestors from herbivorous dinosaurs became an important driver that influenced the development of grazing defense mechanisms. The grazing pressure from herbivorous dinosaurs required ancestral grasses to produce a low growing point below grazing height, to produce double the herbage biomass greater than the leaf area needed for photosynthesis, to develop the structures and hormone systems to greatly improve water use efficiency, to develop a complex system for compensatory physiological growth from meristematic tissue that can rapidly assimilate newly fixed carbon and microbial mineral nitrogen to replace grazed leaf and stem structures, to develop a highly competitive belowground system with symbiotic fungi for uptake of soil water and nutrients, to produce meristematic tissue in axillary buds and a controlling hormone feedback system for vegetative reproduction of tillers, and to shed the ability to produce anthiherbivory toxic substances (Manske 2018).
Modern C3 perennial grasses from the Pooideae subfamily of the BOP clade and grasses with both C3 and C4 photosynthesis from several subfamilies of the PACMAD clade possess these same grazing defense mechanisms which means that the development of these mechanisms must have occurred at a very early stage of ancestral grass during the late Early Cretaceous. The development of these grazing defense mechanisms from grazing pressure by herbivorous dinosaurs permit existent grasslands to be the primary forage source for todays livestock production industry.
Cold Tolerance Mechanisms
Ancestral grasses developed in a world of the late Early Cretaceous that was much warmer than the present and grasses did not need cold tolerance mechanisms. The earth started to become cooler during the late Eocene and the Oligocene, 48 to 26 Ma. The dinosaurs had been gone for at least 20 million years. Antarctica had broken away from Gondwana and was moving to the South Pole. Around 33 Ma, the newly opened Southern Ocean permitted the cold circumpolar currents to cause ocean temperatures to drop. Antarctica became frigid and covered with ice, and the global climate became much colder (Retallack 2004).
Grasses that lived in the Temperate Zones had several million years to develop their cold tolerance mechanisms. All grasses shared a common ancestral trait that prevented cellular dehydration, which was also beneficial in the development of cold acclimation responses.
Enhancement of freezing tolerance was provided by a genetically controlled, Poaceae specific, inhibitor of ice recrystallization, by production of a protein (IRIP), which helps prevent cell rupture by ice crystal development (Preston and Sandve 2013, Korner 2016).
Grasses also developed a seasonal phenological cycle with vernalization responsiveness that allows synchronization of vegetative growth and flowering with favorable conditions of spring and activation of autumnal senescence for termination of growth activity before damaging freezing temperatures. Autumnal senescence permits translocation of synthesized compounds from cells downwards to be stored in the crown (Preston and Sandve 2013, Korner 2016). These changes in spring and fall phenological activities are controlled by the local photoperiod (changes in the length of daylight) which is detected by photoreceptors in specialized plant cells.
Perennial grass plants live for, at least, 27 to 43 years (that is the longest time period that data have been collected). With proper management, grass plants could live for an extremely long time. Each grass tiller developed by vegetative growth of axillary buds lives two growing seasons and one winter dormancy season. The maintenance of life in grass plant cells during the winter period depends on stored carbohydrates that are assimilated during the winter hardening process that occurs during mid August to hard frost. The carbohydrates are used for respiration that provides energy for perpetuation of all necessary crown cells, carryover leaves and stems, and the meristematic tissue until they can be reactivated for growth during the following spring. Grass growth outside of the Tropical Zones would not be possible without the development of the cold tolerance mechanisms.
Lack of Drought or Fire Defense Mechanisms
Perennial grass plants living in temperate zones are known to have relatively high survival rates following environmental stressful conditions of drought and fire. However, perennial grasses have no measurable mechanisms activated by either drought or fire events. These usually high survival rates during drought or fire conditions are attributed to the development of mechanisms that have evolved for grass adaptation to living in dry open habitats, to the defense of defoliation by grazing, and to tolerate cold temperatures.
Grass plants have not developed drought defense mechanisms. When grasses transform into summer dormancy because of the lack of soil water, the grasses do not develop to complete dormancy. Grasses appear to maintain the same quantity of active tissue as they do during winter dormancy. As a result of not being able to reach complete summer dormancy, a greater percent of the grass biomass dies during drought conditions than would not die if they could develop complete dormancy.
Grass plants have not developed fire defense mechanisms. Fire damages, kills, or consumes the aboveground parts of grasses, and if the soil is dry, some of the belowground parts of grasses. Fire recovery processes are deficient as a result from the failure of grass plants to develop fire defense mechanisms. Fire cannot restore degraded grassland ecosystems because fire does not stimulate vegetation reproduction by tillering, fire does not stimulate endomycorrhizal fungal colonization of grass roots, fire does not stimulate rhizosphere organism biomass and activity levels, fire does not stimulate conversion of soil organic nitrogen into inorganic nitrogen, fire does not improve soil water holding capacity, and fire cannot remove woody species that reproduce by vegetative suckers. Repeated fire treatments can modify the composition of the aboveground vegetation in a degraded grassland, but when the fire regime stops, the degraded grassland remains deteriorated. Fire does not improve grassland ecosystems biologically or ecologically, and fire cannot replace the beneficial partial defoliation achieved by grazing graminivores in managing healthy and productive grassland ecosystems.
Internal Grass Growth Mechanisms
The key factor in meeting grass plant biological requirements is proper timing of partial defoliation that removes 25% to 33% of the leaf biomass from grass lead tillers at vegetative growth stages between the 3.5 new leaf stage and the flower (anthesis) stage (1 June to 15 July) that activates the internal grass growth mechanisms that enable grass tillers to withstand and recover from partial defoliation by grazing of large graminivores.
The effects of defoliation are not simply the removal of herbage from grass plants (Langer 1963, 1972): foliage removal disrupts plant growth and photosynthesis, and defoliation also changes physiological mechanisms in all parts of the plant; alters the plant community microclimate by changing light transmission, moisture relations, and temperature (Briske and Richards 1994, 1995); and changes the soil environment, thereby affecting soil organism activity and ecosystem biogeochemical processes (Manske 2000a, 2011a).
Compensatory Physiological Growth
The compensatory physiological growth mechanisms give grass plants the capability to replace lost leaf and shoot biomass following grazing by increasing meristematic tissue activity, increasing photosynthetic capacity, and increasing allocation of carbon and nitrogen (McNaughton 1979, 1983; Briske 1991). Fully activated mechanisms can produce replacement foliage at 140% of the weight that was removed during grazing (Manske 2000b, 2010a, b, 2014a, b). The growth rates of replacement leaves and shoots increase after partial defoliation by grazing. The enhanced activity of meristematic tissue produces larger leaves with greater mass (Langer 1972, Briske and Richards 1995). Developing leaf primordia not fully expanded at time of defoliation have increased growth rates and tend to grow larger than leaves on undefoliated tillers (Langer 1972). Partial defoliated tillers increase photosynthetic rates of remaining mature leaves and rejuvenated portions of older leaves not completely senescent (Atkinson 1986, Briske and Richards 1995). Changes in cytokinin levels and other signals produced as a result of the increase in the root-shoot ratio rejuvenate the photosynthetic apparatus, inhibit or reduce the rate of senescence, and increase the lifespan and leaf mass of remaining mature leaves (Briske and Richards 1995). Activation of the compensatory physiological mechanisms after partial defoliation of grass tillers by grazing requires alternative sources of abundant carbon and nitrogen (Coyne et al. 1995). Carbon fixed during current photosynthesis in remaining mature leaf and shoot tissue and rejuvenated portions of older leaves is preferentially allocated to areas of active meristematic tissue (Ryle and Powell 1975, Richards and Caldwell 1985, Briske and Richards 1995, Coyne et al. 1995). The quantity of leaf area required to fix adequate quantities of carbon is 67% to 75% of the predefoliated leaf area (Manske 1999, 2011a, 2014c). Very little, if any, of the carbon and nitrogen stored in the root system is remobilized to support compensatory growth (Briske and Richards 1995). The mobilizable nitrogen pools in the shoot tissue are reduced following partial defoliation. This loss in nitrogen from the shoot increases preferential use of the quantities of mineral nitrogen available in the media around the roots (Millard et al. 1990, Ourry et al. 1990). This available soil mineral nitrogen has been converted from soil organic nitrogen by active rhizosphere organisms, absorbed through the roots, and moved to areas of active meristematic tissue.
Vegetative Reproduction by Tillering
Vegetative secondary tillers are shoots that develop on lead tillers from growth of axillary buds by the process of tillering (Mueller and Richards 1986, Richards et al. 1988, Murphy and Briske 1992, Briske and Richards 1994, 1995, Dahl 1995). Meristematic activity in axillary buds and the subsequent development of vegetative tillers is regulated by auxin, a growth-inhibiting hormone produced in the apical meristem and young developing leaves (Briske and Richards 1995). Tiller growth from axillary buds is inhibited indirectly by auxin interference with the metabolic function of cytokinin, a growth hormone (Briske and Richards 1995). Partial defoliation of young leaf material at vegetative growth stages temporarily reduces the production of the blockage hormone, auxin (Briske and Richards 1994). This abrupt reduction of plant auxin in the lead tiller allows for cytokinin synthesis or utilization in multiple axillary buds, stimulating the development of vegetative secondary tillers (Murphy and Briske 1992, Briske and Richards 1994). If no defoliation occurs before the flower (anthesis) stage, the lead tiller continues to hormonally inhibit secondary tiller development from axillary buds. Production of the inhibitory hormone, auxin, declines gradationally as the lead tiller reaches the flower stage. The natural reduction of auxin in the lead tiller usually permits only one secondary tiller to develop. This developing secondary tiller produces auxin that hormonally suppress development of additional axillary buds (Briske and Richards 1995). Vegetative tiller growth is the dominant form of reproduction in semiarid and mesic grasslands (Belsky 1992, Chapman and Peat 1992, Briske and Richards 1995, Chapman 1996, Manske 1999) not sexual reproduction and the development of seedlings. Recruitment of new grass plants developed from seedlings is negligible in healthy grassland ecosystems.
Nutrient Resource Uptake
Grass plant dominance within a grassland community is related to the plants competitiveness at nutrient and water resource uptake. Crider (1955) found that grass tillers with 50% or more of the aboveground leaf material removed reduce root growth, root respiration, and root nutrient absorption resulting in reduced functionality of these grass plants. Reduction of active root biomass caused diminishment of grass plant health and vigor (Whitman 1974) that resulted in a loss of resource uptake efficiency and a suppression of the competitiveness of grass plants to take up mineral nitrogen, essential elements, and soil water (Li and Wilson 1998, Kochy 1999, Kochy and Wilson 2000, Peltzer and Kochy 2001). The loss of active root length contributed to the reduction of rhizosphere biomass and the decline of ecosystem biogeochemical processes (Coleman et al. 1983, Klein et al. 1988). The nutrient resource uptake competitiveness of healthy grasses is able to suppress the expansion of shrubs and prevent successful establishment of grass, forb, and shrub seedlings into grasslands (Peltzer and Kochy 2001). The grass growth form has competitive advantages of nutrient uptake over the shrub growth form (Kochy and Wilson 2000). Grass aboveground biomass is primarily productive photosynthetic leaves resulting in a high resource uptake efficiency. Grasses are good competitors for belowground nutrient resources and superior competitors for mineral nitrogen because of a high root: shoot ratio and no woody stems to maintain. Shrubs have a great reduction in resource uptake efficiency because a large portion of the photosynthates produced in the leaves must be used to build and maintain their unproductive woody stems. However, the taller woody stems make shrubs superior competitor for aboveground sunlight resources (Kochy and Wilson 2000). Competition for belowground nutrient resources from healthy grasses reduce the growth rates of shrub rhizomes and cause high mortality rates of young suckers (Li and Wilson 1998). Shrubs can compete for some of the belowground resources only after the grass plants have been degraded by ineffective management. Following the reduction in grass plant resource uptake competitiveness, the belowground resources no longer consumed by the smaller, less vigorous degraded grasses, are taken up by the shrub plants resulting in proportional increases of biomass production (Kochy and Wilson 2000). With greater nutrient resources, shrub rhizome suckers are able to establish a faster growth rate and a higher survival rate (Li and Wilson 1998). The resulting greater shrub stem density increases the competition for the aboveground resources of light causing strong suppression of the grasses (Kochy and Wilson 2000). Traditionally, the observation of increasing woody shrubs and trees into degraded grasslands would have been explained as a result of fire suppression (Humphrey 1962, Stoddart, Smith, and Box 1975, Wright and Bailey 1982).
Water Use Efficiency
Grasslands of the Northern Plains managed with traditional practices are notorious for their inhibitory deficiency in available soil mineral nitrogen (Goetz et al. 1978) which has been determined to cause the observed low herbage production. Deficiencies in mineral nitrogen limit herbage production more often than water in temperate grasslands (Tilman 1990). The total herbage biomass production on grassland ecosystems has been shown to increase with increases in the quantity of available soil mineral nitrogen (Rogler and Lorenz 1957; Whitman 1957, 1963, 1976; Smika et al. 1965; Goetz 1969, 1975; Power and Alessi 1971; Lorenz and Rogler 1972; Taylor 1976; Wight and Black 1979). Greater quantities of available soil mineral nitrogen has been shown to also cause the soil water use efficiency to improve in grassland plants (Smika et al. 1965, Wight and Black 1972, Whitman 1976, 1978). Using a proxy method, Wight and Black (1972) found that precipitation (water) use efficiency of grass plants improved when soil mineral nitrogen was available at threshold quantities of 100 lbs/ac and greater. The inhibitory deficiencies of mineral nitrogen on grasslands that had less than 100 lbs/ac of available soil mineral nitrogen caused the weight of herbage production per inch of precipitation received to be reduced an average of 49.6% below the weight of herbage produced per inch of precipitation on the grassland ecosystems that had greater than 100 lbs/ac of mineral nitrogen and did not have mineral nitrogen deficiencies (Wight and Black 1979). The efficiency of water use in grass plants function at low levels when mineral nitrogen is deficient and function at high levels when mineral nitrogen is available at threshold quantities of 100 lbs/ac or greater. The level of water use efficiency determines the level of herbage biomass productivity on grasslands.
Manske (2010a, b) found that the threshold quantity of 100 lbs/ac of available mineral nitrogen was also critical for functionality of the vegetative reproduction and the compensatory physiological mechanisms. Both these mechanisms function at high potential levels on grasslands that have 100 lbs/ac or greater available soil mineral nitrogen and do not function or function at extremely low levels on grasslands that have mineral nitrogen deficiencies (Manske 2009, 2010a, b, c, 2011b).
Microorganisms of the Rhizosphere
The microbial rhizosphere structure is a cylinder of soil particles bonded by fungal secreted adhesive polysaccharides that surrounds active perennial roots of grassland plants growing in intact soils and the cylinder is inhabited by symbiotic resident organisms and frequent regular visiting organisms. The numerous types of rhizosphere microorganisms are organized along a trophic hierarchy with a means of “communication” among the microbes and with the plant (Manske 2018d). The resident organisms are bacteria, protozoa, and endomycorrhizal fungi and the visiting organisms are nematodes, springtails, and mites (Elliot 1978, Anderson et al. 1981, Harley and Smith 1983, Curl and Truelove 1986, Whipps 1990, Campbell and Greaves 1990) and another resident organism is ectomycorrhizal fungi (Caesar-TonThat et al. 2001b, Manske and Caesar-TonThat 2003, Manske 2007). The numerous types of bacteria have low carbon content. Bacteria are microscopic single celled saprophytic organisms that collectively consume large quantities of soil organic matter and are major primary producers of the rhizosphere. Increases in biomass and activity of the bacteria trophic level elevates the concentration of respiratory carbon dioxide (CO2) resulting in stimulation of activity in the other rhizosphere organisms. Protozoa are single celled microorganisms that are mainly small amoeba in grassland soils and feed primarily on bacteria.
The slightly larger rhizosphere organisms are mobile and move among various rhizosphere structures. Nematodes are a diverse group of small nonsegmented worms. Most nematodes feed primarily on bacteria or fungi, some feed on protozoa, and some eat other nematodes. Springtails are among the most abundant insect in grassland soils that travel among rhizosphere structures. Minute springtails ingest considerable quantities of soil organic matter in order to eat fungi and bacteria. Mites are small eight-legged arachnids that travel among rhizosphere structures and feed on fungi, nematodes, small insects, and other mites. Mites help distribute fungus spores and bacteria through the soil by carrying them on their exoskeleton.
Two types of fungi are resident organisms of the rhizosphere; Endomycorrhizal fungi and Ectomycorrhizal fungi. Endomycorrhizal fungi are also major primary producers of the rhizosphere and are achlorophyllous saprophytes that live on dead organic matter and cannot fix carbon for energy. Endomycorrhizal fungi develop arbuscules, vesicles, and hyphae within root tissue of the host plant (Harley and Smith 1983) and secrete adhesive polysaccharides that bond soil particles around grass roots forming the structural environment for all rhizosphere organisms. The adhesive polysaccharides also bind soil into aggregates resulting in increased soil pore spaces, increased water holding capacity, and increased rooting depth. Endomycorrhizal fungi also move phosphorus, other macro and micro mineral nutrients, and water through the hyphae to the grass roots for absorption (Moorman and Reeves 1979, Harley and Smith 1983, Allen and Allen 1990, Box and Hammond 1990, Marschner 1992, Koide 1993, Marschner and Dell 1994, Smith and Read 1997).
Ectomycorrhizal fungi develop a sheath around the grass root with hyphae that do not enter the tissue of the host plant (Harley and Smith 1983) and secrete large amounts of adhesive polysaccharides forming water-stable aggregates in soil that are water permeable but not water soluable. The increased soil aggregation improves soil quality, increases soil oxygenation, increases water infiltration, and decreases erodibility (Caesar- TonThat and Cochran 2000, Caesar-TonThat et al. 2001a, Caesar-TonThat et al. 2001b, Caesar-TonThat 2002, Manske and Caesar-TonThat 2003, Manske 2007).
The bacteria and fungi are the microflora saprotrophic organisms at the bottom of the food chain and makeup the greatest biomass of the rhizosphere. Both bacteria and fungi contain high proportions of nitrogen in relation to their carbon content. The microfauna trophic level organisms with normal ratios of carbon to nitrogen, consume bacteria or fungi and ingest greater quantities of nitrogen than they need for a balanced diet based on energy (carbon); the excess nitrogen is excreted as ammonium (NH4). The endomycorrhizal fungi can nitrify the excreted ammonium into nitrate (NO3) and pass either form of mineral nitrogen into the grass plant through its endophytic vesicles and arbuscules. The elevated rhizosphere organism activity caused by the increase in available short chain carbon energy exudated from the grass lead tillers following partial defoliation by graminivores results in greater quantities of organic nitrogen mineralized into inorganic nitrogen (Coleman et al. 1983, Klein et al. 1988, Burrows and Pfleger 2002, Rillig et al. 2002, Bird et al. 2002, Driver et al. 2005).
The belowground biogeochemical processes are performed by rhizosphere microorganisms. Grassland soil microflora trophic levels cannot produce their own carbon energy; they lack chlorophyll (achlorophyllous). Also, a large biomass of soil microbes cannot be supported on the small quantities of energy remaining in dead grass roots and leaves. However, grass lead tillers produce large quantities of surplus photosynthate containing short chain carbon energy during vegetative growth stages. This source of carbohydrate energy can be used annually between 1 June and 15 July to feed and sustain a large biomass of rhizosphere microbes (Manske 2018a). The combined weight of the belowground microorganisms on an acre of grassland should be greater than 960 tons/ac (Manske 2023).
Biogeochemical Processes
The indispensable rhizosphere microorganisms are responsible for the performance of the ecosystem nutrient flow activities and for the ecosystem biogeochemcial processes that determine grassland ecosystem productivity and functionality (Manske 2018d).
Biogeochemical processes transform stored essential elements from organic forms or ionic forms into plant usable mineral forms.
Biogeochemical processes capture replacement quantities of lost or removed major essential elements of carbon, hydrogen, nitrogen, and oxygen with assistance from active live plants and transform the replacement essential elements into storage as soil organic matter for later use.
Biogeochemical processes decompose complex unusable organic material into compounds and then into reusable major and minor essential elements (McNaughton 1979, 1983; Coleman et al. 1983; Ingham et al. 1985; Mueller and Richards 1986; Richards et al. 1988; Briske 1991; Murphy and Briske 1992; Briske and Richards 1994, 1995).
The quantity of biogeochemical processes conducted in grassland ecosystems is dependent on the rhizosphere volume and microorganism biomass (Coleman et al. 1983). Rhizosphere volume and microorganism biomass are limited by access to simple carbohydrate energy (Curl and Truelove 1986). Healthy grass plants produce double the quantity of leaf biomass (Crider 1955, Coyne et al. 1995), capture and fix large amounts of carbon during photosynthesis, and produce carbohydrates in quantities greater than the amount needed for normal growth and maintenance (Coyne et al. 1995). Partial defoliation of grass lead tillers at vegetative phenological growth stages by large grazing graminivores causes greater quantities of exudates containing simple carbohydrates to be released from the grass tillers through the roots into the rhizosphere (Hamilton and Frank 2001). With the increase in availability of carbon compounds in the rhizosphere, the biomass and activity of the microorganisms increases (Anderson et al. 1981, Curl and Truelove 1986, Whipps 1990). The increase in rhizosphere organism biomass and activity results in greater quantities of biogeochemical cycling of essential elements (Coleman et al. 1983, Biondini et al. 1988, Klein et al. 1988, Burrows and Pfleger 2002, Rillig et al. 2002, Bird et al. 2002, Driver et al. 2005).
Pestiferous Grasshopper Habitat Management
Proactive preventive pestiferous grasshopper habitat management uses the biologically effective twice-over rotation strategy that was designed to coordinate partial defoliation events with grass phenological growth stages, to meet the nutrient requirements of the grazing graminivores, the biological requirements of the grass plants and the rhizosphere microorganisms, to enhance the ecosystem biogeochemical processes, and to activate the internal grass plant growth mechanisms in order to change grassland ecosystems habitat characteristics to be less favorable for grasshoppers.
The twice-over rotation grazing management strategy uses three to six native grassland pastures. Each pasture is grazed for two periods per growing season. The number of grazing periods is determined by the number of sets of tillers: one set of lead tillers and one set of vegetative secondary tillers per growing season. The first grazing period is 45 days long, ideally, from 1 June to 15 July, with each pasture grazed for 7 to 17 days (never less or more). The number of days of the first grazing period on each pasture is the same percentage of 45 days as the percentage of the total season’s grazeable forage contributed by each pasture to the complete system. The forage is measured as animal unit months (AUM’s). The average grazing season month is 30.5 days long (Manske 2012). The number of days grazed are not counted by calendar dates but by the number of 24-hr periods grazed from the date and time the livestock are turned out to pasture. The second grazing period is 90 days long, ideally from 15 July to 14 October, each pasture is grazed for twice the number of days as in the first period. The length of the total grazing period is best at 135 days; 45 days during the first period plus 90 days during the second period.
There is some flexibility in the grazing period dates. The starting date has a variance of plus or minus 3 days with a range of start dates from 29 May to 4 June. This gives an extreme early option to start on 29 May with the first period to 12 July and with the second period to 11 October. The extreme late alternative option can start on 4 June with the first period to 18 July and with the second period to 17 October. There is also the option to add a total of 2 days to the total length of the grazing period. These 2 days can be used when a scheduled rotation date occurs on an inconvenient date by adding one day to each of two rotation dates. The limit of additional days is two per year resulting in a total length of 137 days. If inconvenient rotation dates occur during 3 or more times, an equal number of days greater than two must be subtracted from the grazing season, so total number of days grazed per year does not exceed 137 days. If the start date is later than 4 June, the scheduled rotation dates must remain as if the start date were on 4 June, in order to maintain the coordinated match of the partial defoliation events with the grass phenological growth stages. The total number of days grazed will be 135 days minus the number of days from 4 June to the actual start date. However, it is best to start on 1 June each year.
During the first period, partial defoliation that removes 25% to 33% of the leaf biomass from grass lead tillers between the 3.5 new leaf stage and the flower stage increases the rhizosphere microbe biomass and activity, enhances the ecosystem biogeochemical processes, and activates the internal grass plant growth mechanisms. Manipulation of these processes and mechanisms does not occur at any other time during a growing season. During the second grazing period, the lead tillers are maturing and declining in nutritional quality and defoliation by grazing is only moderately beneficial to grass development. Adequate forage nutritional quality during the second period depends on the activation of sufficient quantities of vegetative secondary tillers from axillary buds during the first period. Livestock are removed from intact grassland pastures in mid October, towards the end of the perennial grass growing season, in order to allow the carryover tillers to store the carbohydrates and nutrients which will maintain plant mechanisms over the winter. Most of the upright vegetative tillers on grassland ecosystems during the autumn will be carryover tillers which will resume growth as lead tillers during the next growing season. Almost all grass tillers live for two growing seasons, the first season as vegetative secondary tillers and the second season as lead tillers. Grazing carryover tillers after mid October causes the termination of a large proportion of the population, resulting in greatly reduced herbage biomass production in subsequent growing seasons. The pasture grazed first in the rotation sequence is the last pasture grazed during the previous year; i.e. ABC, CAB, and BCA, because the last pasture grazed has the greatest live herbage weight on 1 June of the following season (Manske 2018).
Stocking rates are based on peak herbage biomass on seasonlong grazing practices. The starting stocking rate on the “new” twice-over grazing practice is usually 80% to 100% of the seasonlong stocking rate. It usually requires three grazing seasons with the twice-over strategy stocked at 100% to increase the rhizosphere microbe biomass to be great enough to mineralize 100 lbs/ac of mineral nitrogen (nitrate NO3 and ammonium NH4). After the increased rhizosphere microbe biomass can mineralize 100 lbs/ac of mineral nitrogen, the stocking rate can be increased at 10% per year until the system is stocked at 140% of the seasonlong stocking rate. This has been the maximum biological potential reached on North American grasslands from the twice-over rotation strategy.
Once a rotation date scheduled has been determined, do not change that schedule greater than one day for any worldly reason. If you do not like your neighbors bull, build a fence that the bull cannot jump. If you have water sources that sometimes go dry, put in a water tank system on a pipeline. Fix the problems that develop with solutions that do not change the rotation schedule.
Acknowledgment
I am grateful to Sheri Schneider for assistance in production of this manuscript and development of the tables.
Literature Cited
Allen, E.B., and M.F. Allen. 1990. The mediation of competition by mycorrhizae in successional and patchy environments. p. 307-389. in J.B. Grace and D. Tilman (eds.). Perspectives on plant competition. Academic Press Inc., San Diego, CA.
Anderson, R.V., D.C. Coleman, C.V. Cole, and E.T. Elliott. 1981. Effect of nematodes Acrobeloides sp. and Mesodiplogaster lheritieri on substrate utilization and nitrogen and phosphorus mineralization. Ecology 62:549-555.
Anissimov, M. 2013. What is the evolutionary history of insects? http://www.wisegeek.com.
Atkinson, C.J. 1986. The effect of clipping on net photosynthesis and dark respiration rates of plants from an upland grassland, with reference to carbon partitioning in Festuca ovina. Annals of Botany 58:61-72.
Beard, J.B. 1973. Turfgrass: science and culture. Prentice-Hall, Inc., Englewood Cliffs, NJ.
Bell, C.J., E.L. Lundelius, and 7 coauthors. 2004. The Blancan, Irvingtonian and Rancholabrean Mammal Ages. Chapter 7 in Research Gate. p232-314.
Belovsky, G.E. 2000. Grasshopper population regulation. United States Department of Agriculture, Animal and Plant Health Inspection Service. Grasshopper Integrated Pest Management User Handbook. Technical Bulletin No. 1809. Washington, D.C. p. VII. 14-1-VII. 14-8.
Belsky, A.J. 1992. Effects of grazing competition, disturbance and fire on species composition and diversity grassland communities. Journal of Vegetation Science 3:187-200.
Biondini, M., D.A. Klein, and E.F. Redente. 1988. Carbon and nitrogen losses through root exudation by Agropyron cristatum, A. smithii, and Bouteloua gracilis. Soil Biology and Biochemistry 20:477-482.
Bird, S.B., J.E. Herrick, M.M. Wander, and S.F. Wright. 2002. Spatial heterogeneity of aggregate stability and soil carbon in semi-arid rangeland. Environmental Pollution 116:445-455.
Box, J.E., and L.C. Hammond. 1990. Rhizosphere dynamics. Westview Press, Boulder, CO.
Briske, D.D. 1991. Developmental morphology and physiology of grasses. p. 85-108. in R.K. Heitschmidt and J.W. Stuth (eds.). Grazing management: an ecological perspective. Timber Press, Portland, OR.
Briske, D.D., and J.H. Richards. 1994. Physiological responses of individual plants to grazing: current status and ecological significance. p. 147-176. in M. Vavra, W.A. Laycock, and R.D. Pieper (eds.). Ecological implications of livestock herbivory in the west. Society for Range Management, Denver, CO.
Briske, D.D., and J.H. Richards. 1995. Plant response to defoliation: a physiological, morphological, and demographic evaluation. p. 635-710. in D.J. Bedunah and R.E. Sosebee (eds.). Wildland plants: physiological ecology and developmental morphology. Society for Range Management, Denver, CO.
Burrows, R.L., and F.L. Pfleger. 2002. Arbuscular mycorrhizal fungi respond to increasing plant diversity. Canadian Journal of Botany 80:120-130.
Butler, J.L., and D.D. Briske. 1988. Population structure and tiller demography of the bunch grass Schizachyrium scoparium in response to herbivory. Oikos 51:306-312.
Caesar-TonThat, T.C., and V. Cochran. 2000. Soil aggregate stabilization by a saprophytic lignin-decomposing basidiomycete fungus. I. Microbiological aspects. Biology and Fertility of Soils 32:374-380.
Caesar-TonThat, T.C., W. Shelver, R.G. Thorn, and V.L. Cochran. 2001a. Generation of antibodies for soil aggregating basidiomycete detection to determine soil quality. Applied Soil Ecology 18:99-116.
Caesar-TonThat, T.C., D.H. Branson, J.D. Reeder, and L.L. Manske. 2001b. Soil-aggregating basidiomycetes in the rhizosphere of grasses under two grazing management systems. Poster. American Society of Agronomy Annual Meeting. Charlotte, NC.
Caesar-TonThat, T.C. 2002. Soil binding properties of mucilage produced by a basidiomycete fungus in a model system. Mycological Research 106:930-937.
Campbell, R., and M.P. Greaves. 1990. Anatomy and community structure of the rhizosphere. p. 11-34. in J.M. Lynch (ed.). The rhizosphere. John Wiley and Sons, New York, NY.
Carrathers, R.I., T.S. Larkin, H. Firstencel, and Z. Feng. 1992. Influence of thermal ecology on the mycosis of a rangeland grasshopper. Ecology 73(1):190-204.
Chapman, G.P., and W.E. Peat. 1992. An introduction to the grasses. C.A.B. International, Wallingford, UK. 111p.
Chapman, G.P. 1996. The biology of grasses. C.A.B. International, Wallingford, UK. 273p.
Chintauan-Marquier, I.C., S. Jordan, P. Berthier, and C. Amedegnata. 2011. Evolutionary history and taxonomy of a shorthorned grasshopper subfamily: The Melanoplinae (Orthoptera: Acrididae). Molecular Phylogenetics and Evolution 58:22-32.
Coleman, D.C., C.P.P. Reid, and C.V. Cole. 1983. Biological strategies of nutrient cycling in soil ecosystems. Advances in Ecological Research 13:1-55.
Coyne, P.I., M.J. Trlica, and C.E. Owensby. 1995. Carbon and nitrogen dynamics in range plants. p. 59-167. in D.J. Bedunah and R.E. Sosebee (eds.). Wildland plants: physiological ecology and developmental morphology. Society for Range Management, Denver, CO.
Crider, F.J. 1955. Root-growth stoppage resulting from defoliation of grass. USDA Technical Bulletin 1102.
Curl, E.A., and B. Truelove. 1986. The rhizosphere. Springer-Verlag, New York, NY.
Cushing, W. 1993. Pocket hopper helper: Grasshopper identification aid. USDA, APHIS, GHIPM. Science and Technology. Dickinson, ND. 20p.
Cushing, W. 1996. Hopper helper. United States Department of Agriculture, Animal and Plant Health Inspection Service. Grasshopper Integrated Pest Management User Handbook. Technical Bulletin No. 1809. Washington, D.C. p. VI. 7-1-VI. 7-20.
Cushing, W.J., R.N. Foster, K.C. Reuter, and D. Hirsch. 1996. Seasonal occurrence of common Western North Dakota grasshoppers. United States Department of Agriculture, Animal and Plant Health Inspection Service. Grasshopper Integrated Pest Management User Handbook. Technical Bulletin No. 1809. Washington, D.C. p. VI. 8-1-VI. 8-6.
Dahl, B.E., and D.N. Hyder. 1977. Developmental morphology and management implications. p. 257-290. in R.E. Sosebee (ed.). Rangeland plant physiology. Range Science Series No. 4. Society for Range Management, Denver, CO.
Dahl, B.E. 1995. Developmental morphology of plants. p. 22-58. in D.J. Bedunah and R.E. Sosebee (eds.). Wildland plants: physiological ecology and developmental morphology. Society for Range Management, Denver, CO.
Davidowitz, G. 2013. Grasshoppers. Arizona-Sonora Desert Museum. http://www.desertmuseum.org.
Driver, J.D., W.E. Holben, and M.C. Rillig. 2005. Characterization of glomalin as a hyphal wall component of arbuscular mycorrhizal fungi. Soil Biology and Biochemistry 37:101-106.
Dyke, A.S. 2005. Late quaternary vegetation history of Northern North America based on pollen, macrofossil, and faunal remains. Geographie physique et Quaternaire 59(2-3): 211-262.
Elliot, E.T. 1978. Carbon, nitrogen and phosphorus transformations in gnotobiotic soil microcosms. M.S. Thesis. Colorado State University, Ft. Collins, CO.
Esau, K. 1960. Anatomy of seed plants. Wiley and Sons, New York, NY.
Evans, M.W., and F.O. Grover. 1940. Developmental morphology of the growing point of the shoot and the inflorescence in grasses. Journal of Agricultural Research 61:481-520.
Fisher, J.R., W.P. Kemp, F.B. Pierson, and J.R. Wight. 1996a. Grasshopper egg development: the role of temperature in predicting egg hatch. United States Department of Agriculture, Animal and Plant Health Inspection Service. Grasshopper Integrated Pest Management User Handbook. Technical Bulletin No. 1809. Washington, D.C. p. IV. 2-1-IV. 2-7.
Fisher, J.R., W.P. Kemp, and J.S. Berry. 1996b. Melanoplus sanguinipes Phenology North-South across the western United States. United States Department of Agriculture, Animal and Plant Health Inspection Service. Grasshopper Integrated Pest Management User Handbook. Technical Bulletin No. 1809. Washington, D.C. p. IV. 6-1-IV. 6-6.
Frank, A.B., J.D. Berdahl, and J.F. Karn. 1997. Phyllochron development in cool-season grasses. XVIII International Grassland Congress Poster.
Frank, A.B. 1996. Evaluating grass development for grazing management. Rangelands 18:106-109.
Goetz, H. 1963. Growth and development of native range plants in the mixed prairie of western North Dakota. M. S. Thesis, North Dakota State University, Fargo, ND. 165p.
Goetz, H. 1969. Composition and yields of native grassland sites fertilized at different rates of nitrogen. Journal of Range Management 22:384-390.
Goetz, H. 1975. Availability of nitrogen and other nutrients on four fertilized range sites during the active growing season. Journal of Range Management 28:305-310.
Goetz, H., P.E. Nyren, and D.E. Williams. 1978. Implications of fertilizers in plant community dynamics of Northern Great Plains rangelands. Proceedings of the First International Rangeland Congress. p. 671- 674.
Gomez. R.A., D.C. Lightfoot, and K.B. Miller. 2012. A phylogenetic review of the North American bandwinged grasshopper genus, Encoptolophus Scudder with description of Nebulatettix gen. n. (Orthoptera: Acrididae: Oedipodinae). Insect Systematics and Evolution 43:117-145.
Hamilton, E.W., and D.A. Frank. 2001. Can plants stimulate soil microbes and their own nutrient supply? Evidence from a grazing tolerant grass. Ecology 82:2397-2402.
Harley, J.L., and S.E. Smith. 1983. Mycorrhizal symbiosis. Academic Press, New York, NY.
Hewitt, G.B., and J.A. Onsager. 1982a. A method for forecasting potential losses from grasshopper feeding on northern mixed prairie forages. Journal of Range Management 35(1):53-57.
Hibbard, C.W. 1958. Summary of North American Pleistocene mammalian local faunas. Michigan Academy of Science. Vol.43:1- 10.
Humphrey, R.R. 1962. Range Ecology. The Ronald Press Co. New York, NY. 234p.
Hyder, D.N. 1974. Morphogenesis and management of perennial grasses in the U.S. p. 89-98. in Plant morphogenesis as the basis for scientific management for range resources. USDA Miscellaneous Publication 1271.
Ingham, R.E., J.A. Trofymow, E.R. Ingham, and D.C. Coleman. 1985. Interactions of bacteria, fungi, and the nemotode grazers: effects of nutrient cycling and plant growth. Ecological Monographs 55:119-140.
Jech, L. 1996. What, when, and where do grasshoppers eat? United States Department of Agriculture, Animal and Plant Health Inspection Service. Grasshopper Integrated Pest Management User Handbook. Technical Bulletin No. 1809. Washington, D.C. p. II. 13-1-II. 13-2.
Joern, A. 1996a. Host plant quality and grasshopper populations. United States Department of Agriculture, Animal and Plant Health Inspection Service. Grasshopper Integrated Pest Management User Handbook. Technical Bulletin No. 1809. Washington, D.C. p. IV. 4-1-IV. 4-6.
Joern, A. 1996b. Nutritional needs and control of feeding. United States Department of Agriculture, Animal and Plant Health Inspection Service. Grasshopper Integrated Pest Management User Handbook. Technical Bulletin No. 1809. Washington, D.C. p. IV. 7-1-IV. 7-8.
Klein, D.A., B.A. Frederick, M. Biondini, and M.J. Trlica. 1988. Rhizosphere microorganism effects on soluble amino acids, sugars, and organic acids in the root zone of Agropyron cristatum, A. smithii, and Bouteloua gracilis. Plant and Soil 110:19-25.
Kochy, M., and S.D. Wilson. 2000. Competitive effects of shrubs and grasses in prairie. Oikos 91:385-395.
Kochy, M. 1999. Grass-tree interactions in western Canada. Ph.D. Dissertation. University of Regina. Regina, Saskatchewan, Canada.
Koide, R.T. 1993. Physiology of the mycorrhizal plant. p. 33-54. in D.S. Ingram and P.H. Williams (eds.). Mycorrhiza synthesis. Academic Press, London, UK.
Korner, C. 2016. Plant adaptation to cold climates. Fl000 Research. 7p.
Langer, R.H.M. 1956. Growth and nutrition of timothy (Phleum pratense). I. The life history of individual tillers. Annals of Applied Biology 44:166-187.
Langer, R.H.M. 1963. Tillering in herbage grasses. Herbage Abstracts 33:141-148.
Langer, R.H.M. 1972. How grasses grow. Edward Arnold, London, Great Britain.
Leopold, A.C., and P.E. Kriedemann. 1975. Plant growth and development. McGraw-Hill Book Co., New York, NY.
Li, X., and S.D. Wilson. 1998. Facilitation among woody plants establishing in an old field. Ecology 79:2694-2705.
Lorenz, R.J., and G.A. Rogler. 1972. Forage production and botanical composition of mixed prairie as influenced by nitrogen and phosphorus fertilization. Agronomy Journal 64:244-249.
Manske, L.L. 1993. Modification of vegetation by grazing and mowing to affect grasshopper populations. USDA/APHIS/PPQ Cooperative Grasshopper Integrated Pest Management Project Annual Report, FY 1993. USDA/APHIS. Boise, ID. p. 81-89.
Manske, L.L. 1994a. Modifications of native range vegetation by grazing management to affect grasshopper populations. USDA/APHIS/PPQ Cooperative Grasshopper Integrated Pest Management Project Annual Report, FY 1994. USDA/APHIS. Boise, ID. p. 99-108.
Manske, L.L. 1999. Can native prairie be sustained under livestock grazing? Provincial Museum of Alberta. Natural History Occasional Paper No. 24. Edmonton, AB. p. 99-108.
anske, L.L. 2000a. Management of Northern Great Plains prairie based on biological requirements of the plants. NDSU Dickinson Research Extension Center. Range Science Report DREC 00-1028. Dickinson, ND. 12p.
Manske, L.L. 2000b. Grazing before grass is ready. NDSU Dickinson Research Extension Center. Range Management Report DREC 00-1032. Dickinson, ND. 6p.
Manske, L.L. and T.C. Caesar-TonThat. 2003. Increasing rhizosphere fungi and improving soil quality with biologically effective grazing management. NDSU Dickinson Research Extension Center. Summary Range Research Report DREC 03-3025. Dickinson, ND. 6p.
Manske, L.L. 2007. Ectomycorrhizal Basidiomycete fungi detected in rhizospheres of mixed grass prairie grasses. NDSU Dickinson Research Extension Center. Summary Range Research Report DREC 07-3047. Dickinson, ND. 3p.
Manske, L.L. 2008a. Annual nutritional quality curves for graminoids in the Northern Plains. NDSU Dickinson Research Extension Center. Summary Range Management Report DREC 08-3014c. Dickinson, ND. 15p.
Manske, L.L. 2008b. Annual mineral quality curves for graminoids in the Northern Plains. NDSU Dickinson Research Extension Center. Range Management Report DREC 08-1030b. Dickinson, ND. 15p.
Manske, L.L. 2009. Grass plant responses to defoliation. NDSU Dickinson Research Extension Center. Range Research Report DREC 09-1074. Dickinson, ND. 47p.
Manske, L.L. 2010a. Leaf stage development of western wheatgrass tillers. NDSU Dickinson Research Extension Center. Range Research Report DREC 10-1075. Dickinson, ND. 48p.
Manske, L.L. 2010b. Evaluation of the defoliation resistance mechanisms influence on vegetative tiller initiation and tiller density. NDSU Dickinson Research Extension Center. Range Research Report DREC 10- 1076. Dickinson, ND. 13p.
Manske, L.L. 2010c. Long-term plant species shift caused by nitrogen fertilization of native rangeland. NDSU Dickinson Research Extension Center. Summary Range Research Report DREC 10-3055. Dickinson, ND. 16p.
Manske, L.L. 2011a. Biology of defoliation by grazing. NDSU Dickinson Research Extension Center. Range Management Report DREC 11-1067b. Dickinson, ND. 25p.
Manske, L.L. 2011b. Soil mineral nitrogen increased above the threshold quantity of 100 pounds per acre in rangeland ecosystems. NDSU Dickinson Research Extension Center. Summary Range Management Report DREC 11-3056. Dickinson, ND. 8p.
Manske, L.L. 2012. Length of the average grazing season month. NDSU Dickinson Research Extension Center. Range Management Report DREC 12-1021c. Dickinson, ND. 2p.
Manske, L.L. 2014a. Grass vegetative tillering responses to partial defoliation. NDSU Dickinson Research Extension Center. Range Research Report DREC 14-1086. Dickinson, ND. 35p.
Manske, L.L. 2014b. Vegetative forage tiller development in response to partial defoliation. NDSU Dickinson Research Extension Center. Range Research Report DREC 14-1087. Dickinson, ND. 26p.
Manske, L.L. 2014c. Grazingland management based on native rangeland ecosystem mechanisms and processes. NDSU Dickinson Research Extension Center. Summary Range Management Report DREC 14-3062. Dickinson, ND. 18p.
Manske, L.L. 2014. Proactive management of pestiferous rangeland grasshopper habitat of the Northern Plains. NDSU Dickinson Research Extension Center. Rangeland Outreach Program DREC 14-4021. Dickinson, ND. 166p.
Manske, L.L. 2018. Restoring degraded grasslands. pp. 325-351. in Marshall, A. and Collins, R. (ed.). Improving grassland and pasture management in temperate agriculture. Burleigh Dodds Science Publishing, Cambridge, UK.
Manske, L.L. 2018. Advanced pasture forage management technology for the Northern Mixed Grass Prairie. NDSU Dickinson Research Extension Center. Rangeland Research Outreach Program DREC 18-4032. Dickinson, ND. 55p.
Manske, L.L. 2018d. Rhizosphere Organisms: An indispensable biotic component of the Northern Mixed Grass Prairie. NDSU Dickinson Research Extension Center. Rangeland Research Outreach Program DREC 18-4030. Dickinson, ND. 91p.
Manske, L.L. 2021. Biologically effective management of Northern Plains grasslands using ecologically scientific principles. NDSU Dickinson Research Extension Center. Range Research Report DREC 21-6000. Dickinson, ND. 92p.
Manske, L.L. 2022. The age of grasses has recently doubled to 113 million years. NDSU Dickinson Research Extension Center. Range Research Report DREC 22-1198. Dickinson, ND. 17p.
Manske, L.L. 2023. How much soil microbial biomass does a healthy grassland need. Range Research Update 23-026.
Marschner, H. 1992. Nutrient dynamics at the soil- root interface (Rhizosphere). p. 3-12. in D.J. Read, D.H. Lewis, A.H. Fitter, and I.J. Alexander (eds.). Mycorrhizas in ecosystems. C.A.B. International, Wallingford, U.K.
Marschner, H., and B. Dell. 1994. Nutrient uptake in mycorrhizal symbiosis. Plant and Soil 159:89-102.
McMillan, C. 1957. Nature of the plant community. III. Flowering behavior within two grassland communities under reciprocal transplanting. American Journal of Botany 44:144-153.
McNaughton, S.J. 1979. Grazing as an optimization process: grass-ungulate relationships in the Serengeti. American Naturalist 113:691-703
McNaughton, S.J. 1983. Compensatory plant growth as a response to herbivory. Oikos 40:329-336.
Millard, P., R.J. Thomas, and S.T. Buckland. 1990. Nitrogen supply affects the remobilization of nitrogen forthe growth of defoliation Lolium perenne L.J. Experimental Botany 41:941-947.
Moorman, T., and F.B. Reeves. 1979. The role of endomycorrhizae in revegetation practices in the semi-arid west. II. A bioassay to determine the effect of land disturbance on endomycorrhizal populations. American Journal of Botany 66:14-18.
Mueller, R.J., and J.H. Richards. 1986. Morphological analysis of tillering in Agropyron spicatum and Agropyron desertorum. Annals of Botany 58:911-921.
Mulkern, G.B., K.P. Pruess, H. Knutson, A.F. Hagen, J.B. Campbell, and J.D. Lambley. 1969. Food habits and preferences of grassland grasshoppers of the North Central Great Plains. North Dakota State University, Agricultural Experiment Station. Bulletin 481. Fargo, ND. 32p.
Murphy, J.S., and D.D. Briske. 1992. Regulation of tillering by apical dominance: chronology, interpretive value, and current perspectives. Journal of Range Management 45:419-429.
Onsager, J.A. and J.E. Henry. 1977. A method for estimating the density of rangeland grasshoppers (Orthoptera: Acrididae) in experimental plots. Acrida. 6:231-237.
Onsager, J.A. 1987. Current tactics for suppression of grasshoppers on range p.60-66. in J.A. Onsager (ed.). Integrated pest management on rangeland: State of the art in the sagebrush ecosystem. United States Department of Agriculture, Agricultural Research Service, ARS-50. Springfield, VA. 85p.
Onsager, J.A. 1996. The importance of grazing strategies to grasshopper management. United States Department of Agriculture, Animal and Plant Health Inspection Service. Grasshopper Integrated Pest Management User Handbook. Technical Bulletin No. 1809, Washington, D.C. p. V.1-1-V.1-3.
Onsager, J.A. 1998. Theoretical benefits of modifying grasshopper life history parameters. Proceedings of the National Grasshopper Management Board 1998 Annual Meeting. Denver, CO. p.3-4.
Onsager, J.A. 2000. Suppression of grasshoppers in the Great Plains through grazing management. Journal of Range Management 53(6):592-602.
Osborne, C.P. 2008. Atmosphere, ecology, and evolution: what drove the Miocene expansion of C4 grasslands? Journal of Ecology 96:35-45.
Ourry, A., J. Boucaud, and J. Salette. 1990. Partitioning and remobilization of nitrogen during regrowth in nitrogen-deficient ryegrass. Crop Science 30:1251-1254.
Parker, M.A. 1982. Thermoregulation by diurnal movement in the barberpole grasshopper (Dactylotum bicolor). The American Midland Naturalist 107(2):228-237.
Peltzer, D.A., and M. Kochy. 2001. Competitive effects of grasses and woody plants in mixed grass prairie. Journal of Ecology 89:519-527.
Pfadt, R.E. 1994. Field guide to common western grasshoppers. 2nd Ed. Wyoming Agricultural Experiment Station Bulletin 912. University of Wyoming. Laramie, WY.
Poinar, G.O. 2004. Programinis burmitis gen. et sp. nov. and P. laminatus sp. nov., Early Cretaceous grass-like monocots in Burmese amber. Australian Systematic Botany 17:497-504.
Poinar, G.O. 2011. Silica bodies in the Early Cretaceous Programinis laminatus (Angiospermae: Poales). Palaeodiversity 4:1-6.
Power, J.F., and J. Alessi. 1971. Nitrogen fertilization of semiarid grasslands: plant growth and soil mineral N levels. Agronomy Journal 63:277-280.
Prasad, V., C.A.E. Stromberg, H. Alimohammadian, and A. Sahni. 2005. Dinosaur coprolites and the early evolution of grasses and grazers. Science 310:1177- 1180.
Prasad, V. and 8 coauthors. 2011. Late Cretaceous origin of the rice tribe provides evidence for early diversification in Poaceae. Nature Communications. 1-9.
Preston, J.C., and S.R. Sandve. 2013. Adaptation to seasonality and the winter freeze. Plant Science. 4(167) 1-18.
Prothero, D.R. 2006. After the dinosaurs. Indiana University Press. Indianapolis, Indiana. 362p.
Rechenthin, C.A. 1956. Elementary morphology of grass growth and how it affects utilization. Journal of Range Management 9:167-170.
Retallack, G.J. 2004. Late Oligocene bunch grassland and early Miocene sod grassland paleosols from central Oregon, USA. Palaeogeography, Palaeoclimatology, Palaeoecology. 207:203-237.
Richards, J.H., and M.M. Caldwell. 1985. Soluble carbohydrates, concurrent photosynthesis and efficiency in regrowth following defoliation: a field study with Agropyron species. Journal of Applied Ecology 22:907-920.
Richards, J.H., R.J. Mueller, and J.J. Mott. 1988. Tillering in tussock grasses in relation to defoliation and apical bud removal. Annals of Botany 62:173-179.
Rillig, M.C., S.F. Wright, and V.T. Eviner. 2002. The role of arbuscular mycorrhizal fungi and glomalin in soil aggregation: comparing effects of five plant species. Plant and Soil 238:325-333.
Roberts, R.M. 1939. Further studies of the effects of temperature and other environmental factors upon the photoperiodic response of plants. Journal of Agricultural Research 59:699-709.
Rogler, G.A., and R.J. Lorenz. 1957. Nitrogen fertilization of Northern Great Plains rangelands. Journal of Range Management 10:156-160.
Ryle, G.J., and C.E. Powell. 1975. Defoliation and regrowth in the graminaceous plant: the role of current assimilate. Annals of Botany 39:297-310.
Sedivec, K. 1999. Nutritional quality of selected rangeland plants. Summary Report. NDSU Animal and Range Sciences Department. Research Report. Fargo, ND.
Semprebon, G.M., F. Rivals, and C.M. Janis. 2019. The role of grass vs. exogenous abrasives in the paleodietary patterns of North American ungulates. Ecology and Evolution. 7(65) 1-23.
Smika, D.E., H.J. Haas, and J.F. Power. 1965. Effects of moisture and nitrogen fertilizer on growth and water use by native grass. Agronomy Journal 57:483-486.
Smith, S.E., and D.J. Read. 1997. Mycorrhizal symbiosis. Academic Press, San Diego, CA.
Stebbins, G.L. 1981. Coevolution of grasses and herbivores. Annals of the Missouri Botanical Garden 68(1) 75-86.
Stoddart, L.A., A.D. Smith, and T.W. Box. 1975. Range Management. 3rd ed. McGraw-Hill Book Co. New York, NY. 532p.
Taylor, J.E. 1976. Long-term responses of mixed prairie rangeland to nitrogen fertilization and range pitting. Ph.D. Thesis, North Dakota State University, Fargo, ND. 97p.
Tilman, D. 1990. Constraints and tradeoffs: toward a predictive theory of competition and succession. Oikos 58:3-15.
Wang, Y., and Z-H. Chen. 2020. Does molecular and structural evolution shape the speedy grass stomata? Frontiers in Plant Science. 21p.
Watts, J.G., G.B. Hewitt, E.W. Huddleston, H.G. Kinzer, R.J. Lavigne, and D.N. Ueckert. 1989. Rangeland entomology. Range Science Series No. 2. 2nd Ed. Society for Range Management. Denver, CO. 388p.
Whipps, J.M. 1990. Carbon economy. p. 59-97. in J.M. Lynch (ed.). The rhizosphere. John Wiley and Sons, New York, NY.
Whitman, W.C., D.W. Bolin, E.W. Klosterman, H.J. Klostermann, K.D. Ford, L. Moomaw, D.G. Hoag, and M.L. Buchanan. 1951. Carotene, protein, and phosphorus in range and tame grasses of western North Dakota. North Dakota Agricultural Experiment Station. Bulletin 370. Fargo, ND. 55p.
Whitman, W.C. 1957. Influence of nitrogen fertilizer on native grass production. Annual Report. Dickinson Experiment Station. Dickinson, ND. p. 16-18.
Whitman, W.C. 1963. Fertilizer on native grass. Annual Report. Dickinson Experiment Station. Dickinson, ND. p. 28-34.
Whitman, W.C. 1974. Influence of grazing on the microclimate of mixed grass prairie. p. 207-218. in Plant Morphogenesis as the basis for scientific management of range resources. USDA Miscellaneous Publication 1271. Berkley, CA.
Whitman, W.C. 1976. Native range fertilization and interseeding studies. Annual Report. Dickinson Experiment Station. Dickinson, ND. p. 11-17.
Whitman, W.C. 1978. Fertilization of native mixed prairie in western North Dakota. Annual Report. Dickinson Experiment Station. Dickinson, ND. p. 20-22.
Wight, J.R., and A.L. Black. 1972. Energy fixation and precipitation use efficiency in a fertilized rangeland ecosystem of the Northern Great Plains. Journal of Range Management 25:376-380.
Wight, J.R., and A.L. Black. 1979. Range fertilization: plant response and water use. Journal of Range Managment 32:345-349.
Wilson, A.M., and D.D. Briske. 1979. Seminal and adventitious root growth of blue grama seedlings on the central plains. Journal of Range Management 32:209-213.
Wright, H.A., and A.W. Bailey. 1982. Fire Ecology: United States and southern Canada. John Wiley & Sons. New York, NY. 501p.
Wu, Y., H-L. You, and X-Q. Li. 2018. Dinosaur associated Poaceae epidermis and phytoliths from the Early Cretaceous of China. National Science Review. 5:721-727.
Agronomy Research
Soybean Phosphorus Impacts on Spring Wheat
Chris Augustin
Center-Director, Soil Scientist Dickinson Research Extension Center
chris.augustin@ndsu.edu; 701-456-1103
Introduction
Soybeans have been found to inconsistently respond to phosphorus fertilizers (Bardella, 2016). However, many fertilize soybeans with phosphorus to prevent a yield or quality drag next to year’s crop. A soybean phosphorus rate study was initiated in 2023 (Augustin, 2023) where triple-superphosphate was hand applied at 0, 23, 46, 69, and 92 lbs P2O5/ac. Those treatments did not impact soybean yield or quality (Augustin, 2023). Spring phosphorus treatments did not increase fall Olsen (0-6 inch depth) soil tests (Figure 1).
Methods Spring wheat (ND Heron) was planted into the 2023 soybean phosphorus plots on May 7, 2024 at 1.1 million pure live seeds per acre. Nitrogen and potassium were broadcasted after planting. The crop was managed following integrated pest management guidelines and harvested with a plot combine. Plots were 5x35 feet.
Results 2023 fertilizer treatments on soybeans did not impact 2024 spring wheat yields (p-value 0.346; C.V. 9.93), protein (p-value 0.951; C.V. 2.37), or test weight (p-value 0.844; C.V. 1.24). Means and ranges for yield, protein, and test weight are in Table 1. The lack of a response could be due to the dry growing season paired with banded/in-furrow phosphorus fertilizers have been found to improve spring wheat yields (Alessi and Power, 1980). Wheat yield and quality was below the long-term average at the Dickinson Research Extension Center (Table 1).
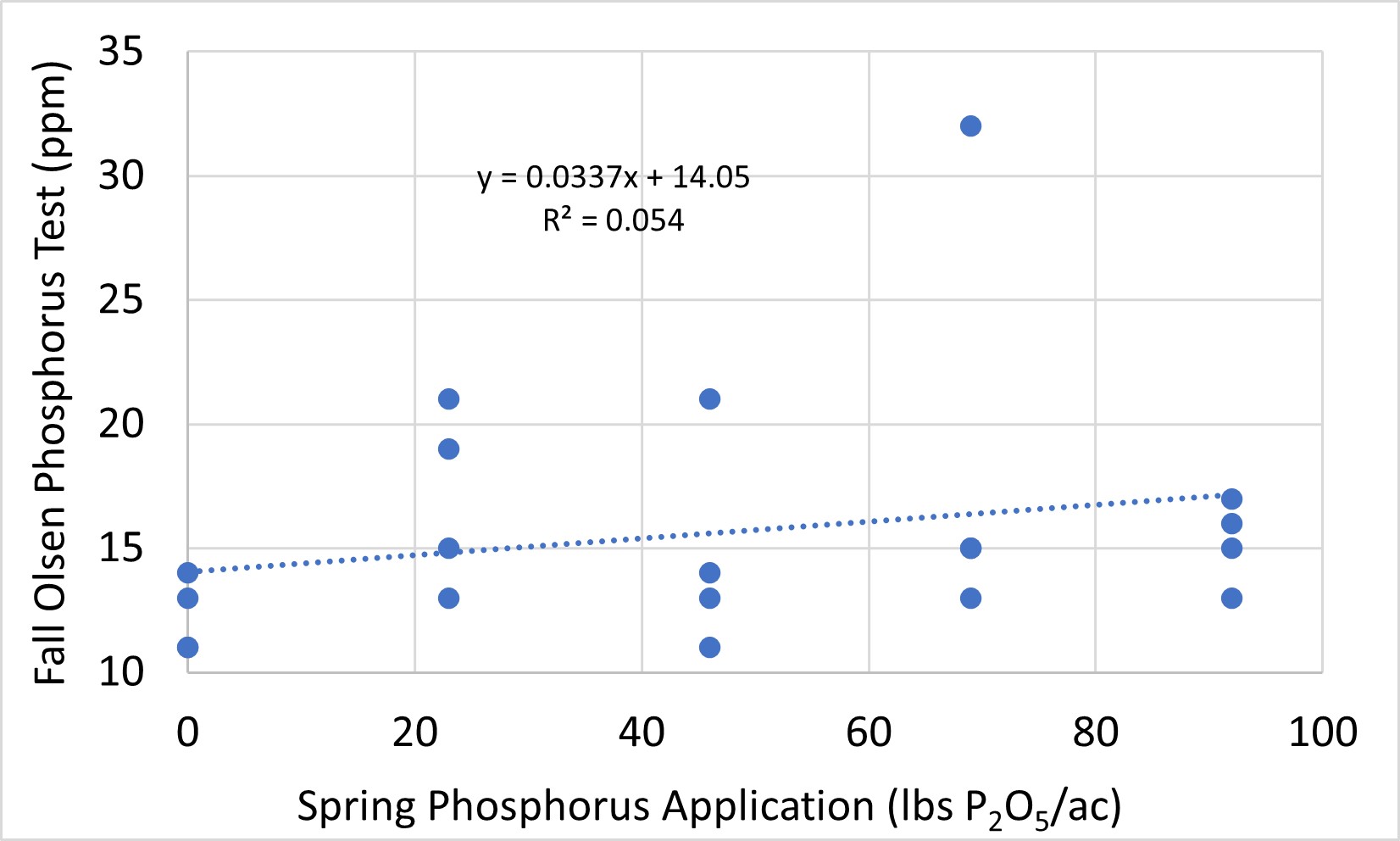
Table 1. Spring wheat yield and quality. | |||
---|---|---|---|
Bushels/Acre | Protein % | Test Weight (lbs/bu) | |
Mean | 25.5 | 18.2 | 48.6 |
Range | 19.4 - 30.7 | 17.8 - 18.5 | 46.9 - 50.2 |
References
Alessi, J., and J.F. Power. 1980. Effects of banded and residual fertilizer phosphorus on dryland spring wheat yield in the Northern Plains. Soil Sci. Soc. Am. J. 44:792-796.
Augustin, C.L. 2023. Phosphorus fertilizer impacts on soybean yield. p 67 In Dickinson Research Extension Center 2023 annual report. NDSU Dickinson Research Extension Center, Dickinson, ND.
Bardella, G.R. 2016. Phosphorus management practices for soybean production in Manitoba. M.S. Thesis. University of Manitoba, Winnipeg, MB.
Sulfur Impacts on Spring Wheat
Chris Augustin
Center-Director, Soil Scientist Dickinson Research Extension Center
chris.augustin@ndsu.edu; 701-456-1103
Introduction
Sulfur fertilizer seems to get more attention each year as the frequency of deficiencies have been increasing. Sulfur is tough to manage (Franzen, 2023a). Small grain fields that are sandy and/or have less than 2% organic matter can benefit from 10 lbs sulfate/ac (Franzen, 2023b). Sulfate forms of fertilizer tend to be the most reliable (i.e. ammonia sulfate). Whereas, elemental sulfur takes time to mineralize and become plant available (Franzen, 2023c). Elemental sulfur may not be rendered plant available to short season crops like canola or wheat when the sulfate demand is greatest.
Methods
This project evaluated sulfur fertilizer use on canola the prior year (2023) and spring applied sulfur (2024) on the current spring wheat crop. Fertilizer was hand applied at 0, 5, 10, 15, 20 lbs sulfur/ac as gypsum, ammonium sulfate, and elemental sulfur the day after planting. A broadcast fertilizer spreader applied 100 lbs nitrogen/ac on the plots. The spring wheat (ND Heron) was planted at 1.1 million pure live seeds per acre on May 13, 2024. The crop was managed following integrated pest management guidelines and harvested with a plot combine. Plots were 5x30 feet.
Results
Sulfur treatments did not improve spring wheat grain yield (p-value 0.514; C.V. 18.60), protein (p-value 0.1651; C.V. 4.68), or test weight (p-value 0.696; C.V. 2.46). The average yield, test weight, a protein content were 24 bu/ac, 47.8 lbs/bu, and 17.1 % respectively. The wheat crop was drought stressed as the wheat grains were shriveled and dull. We will continue this research in 2025 as it is difficult to draw conclusions from one year of research.
References
Franzen, D.W. 2023a. Limitations of the sulfate-sulfur soil test as a predictor of sulfur response SF1880. North Dakota State University Extension, Fargo, ND.
Franzen, D.W. 2023b. North Dakota fertilizer recommendation tables and equations SF882. North Dakota State University Extension, Fargo, ND.
Franzen, D.W. 2023c. Fertilizing canola and mustard SF1122. North Dakota State University Extension, Fargo, ND.
Canola Sulfur Impacts on Spring Wheat Chris Augustin
Center-Director, Soil Scientist Dickinson Research Extension Center
chris.augustin@ndsu.edu; 701-456-1103
Introduction
A canola sulfur trial was conducted in 2023 (Augustin, 2023). Sulfate forms of sulfur are generally recommended as they are more readily plant available and more likely to provide a response for the current crop (Franzen, 2023a). However, some apply elemental sulfur for the following crop. This is difficult to assess since the standard sulfate test can be unreliable and not diagnostic (Franzen, 2023b). This project evaluated spring wheat impacts from the 2023 canola sulfur trial.
Methods
Sulfur was applied to canola in 2023 at rates of 0, 10, 20, and 30 lbs sulfate/ac. Fertilizers used were gypsum, ammonium sulfate, and elemental sulfur. The spring wheat (ND Heron) was planted at 1.1 million pure live seeds per acre on May 15, 2024. The crop was managed following integrated pest management guidelines and harvested with a plot combine. Plots were 5x30 feet.
Results
Treatments did not impact wheat grain yield (p-value 0.292; C.V. 7.57), test weight (p-value 0.481; C.V. 2.66), and protein content (p-value 0.345; C.V. 7.18). Average grain yield, test weight, and protein content were 23 bu/ac, 57.1 lbs/bu, and 14.2 % respectively. The droughty conditions negatively impacted the spring wheat. This project will be redone in 2025 due to the difficulty to develop conclusions from one year of data.
References
Augustin, C.L. 2023. Sulfate fertility impacts on canola grown in southwest North Dakota. p 68 In Dickinson Research Extension Center 2023 annual report. NDSU Dickinson Research Extension Center, Dickinson, ND.
Franzen, D.W. 2023a. Fertilizing canola and mustard SF1122. North Dakota State University Extension, Fargo, ND.
Franzen, D.W. 2023b. Limitations of the sulfate-sulfur soil test as a predictor of sulfur response SF1880. North Dakota State University Extension, Fargo, ND.
Boron Impacts on Canola Chris Augustin
Center-Director, Soil Scientist Dickinson Research Extension Center
chris.augustin@ndsu.edu; 701-456-1103
Boron has gained interest as a micro-nutrient for canola and other crops. Boron is needed for cell walls, hormone regulation, pollination, and seed production. Boron was hand applied at 0, 5, and 10 lbs B/ac after planting. The initial boron soil test was 0.3 ppm. Treatments did not impact canola yield (p-value 0.164; C.V. 35.91). The crop was a failure as the average canola seed yield was 393 lbs/ac and the coefficient of variation (C.V.) was relatively high and suggests that the data was not good. This research will be redone in 2025.
Phosphorus Impacts on Soybean Grown in Southwest North Dakota Chris Augustin
Center-Director, Soil Scientist Dickinson Research Extension Center
chris.augustin@ndsu.edu; 701-456-1103
Introduction
Soybean is an expanding crop in southwestern North Dakota and little data is available to guide soybean farmers on fertilizer management. Soybean has been found to inconsistently respond positively to phosphorus fertilizers in potentially higher yielding environments (Bardella, 2016; Lauzona and Miller, 2008; Mallarino and Borges, 2000; Mallarino and Haq, 2005; Slaton et al., 2010).
Methods
A field trial that evaluated hand applied triple-superphosphate at rates of 0, 23, 46, 69, and 92 lbs P2O5/ac. This research occurred at the Dickinson Research Extension Center (DREC) and nearby Beulah. The previous crop was forage-oat hay and barley at the DREC and Beulah sites respectively. Initial soil tests at the DREC were 3 ppm Olsen phosphorus, pH 6.9, and 64 lbs nitrogen/ac. Soybeans were solid seeded with a no-till drill. Beulah initial soil tests were 2 ppm Olsen phosphorus, pH 6.8, and 41 lbs nitrogen/ac and planted with a hoe-drill air-seeder.
Average yield, protein, and oil content are reported in Table 1. Phosphorus treatments did not impact soybean yield (p-value 0.421; C.V. 26.15), protein (p-value 0.648; C.V. 6.78), and oil (p-value 0.620; C.V. 3.59) content at the DREC site. Phosphorus treatments did not impact yield (p-value 0.065; C.V. 41.73) and protein (p-value 0.135; C.V. 1.69) at the Beulah experiment. However, phosphorus did impact soybean oil content (p-value 0.047; C.V. 1.82). The impact resulted in the lowest oil content from the 69 lbs P2O5/ac treatment.
Results
Phosphorus treatments did not impact soybean grain yields. However, fall soil tests were impacted as both sites had p-values of <0.001 (Figure 1). Phosphorus applications may not impact soybeans, but could impact the subsequent crop. Next year, the DREC site will be planted with spring wheat to see if the previous year’s phosphorus treatment could impact the following year’s wheat crop.
Table 1. Average soybean yield, protein, and oil. | |||
---|---|---|---|
Yield | Protein | Oil | |
-bu/ac- | ---%--- | ||
DREC | 22.0 | 18.9 | 27.7 |
Beulah | 10.0 | 18.0 | 33.4 |
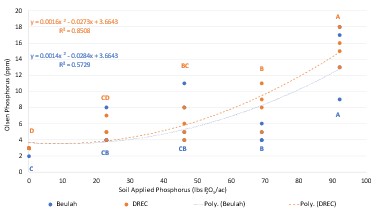
Figure 1. Spring applied phosphorus impacts on fall soil test (p-value <0.001). Different letters indicate statistical significance at the 0.05 level.
References
Bardella, G.R. 2016. Phosphorus management practices for soybean production in Manitoba. M.S. Thesis. University of Manitoba, Winnipeg, MB.
Lauzon, J. D. and Miller, M. H. 1997. Comparative response of corn and soybean to seed‐placed phosphorus over a range of soil test phosphorus. Communications in Soil Sci. and Plant Anal. 28: 205–215.
Mallarino, A.P. and Borges, R. 2000. Grain yield, early growth, and nutrient uptake of no-till soybean as affected by phosphorus and potassium placement. Agron. J. 92: 380-388.
Mallarino, A.P. and Haq, U.M. 2005. Response of soybean grain oil and protein concentrations to foliar and soil fertilization. Agron. J. 97: 910–918.
Slaton, N.A., DeLong, R.E., Shafer, J., Clark, S. and Golden, B.R. 2010. Soybean response to phosphorus and potassium fertilization rate and time. In: N.A. Slaton, ed. Wayne E. Sabbe Arkansas Soil Fertility Studies 2010, University of Arkansas Agricultural Experiment Station Research Series 588:34-37. Fayetteville, Ark.
Crop and Pest Reports
Victor Gomes
Extension Cropping Systems Specialist
Dickinson Research and Extension Center
701-456-1102, victor.gomes@ndsu.edu
May 16, 2024
Southwest North Dakota
Last week, we received between 0.1 to 0.7 inches of rain, causing some delays in activities. In drier areas, there was action mid-week, while moister areas saw activity starting on Saturday, although with reports of machinery moving big chunks of mud. This week, we’ll likely see rain and windy conditions (but less frequent) that could further stall progress.
Crop-wise, we're over 80% planted with small grains, with some of the earlier planted crops emerging well. Canola planting is nearly complete, as the last date for insurance is drawing near. Pulses and soybean planting is starting to pick up and sunflower planting has not quite yet started.
Growers should be on the lookout and scout their fields for alfalfa weevil in Southwest North Dakota, as we have had enough degree days for them to start emerging.
May 23, 2024
Southwest North Dakota
Rainfall events over the past week were brief and scattered. We received between 0.1 inches in Bowman and Dunn counties and 0.3 inches in Adams and Oliver counties. Some areas in the Southwest region, including Golden Valley, Slope, and Bowman counties, are now experiencing moderate drought conditions.
With most small grains now planted, the timely application of post-emergence herbicide is crucial. Even if your preemergent herbicide application was initially successful in reducing weed populations, completing the herbicide program with a timely post-emergence application is essential.
In our region, some canola crops have already emerged, leading to significant flea beetle activity. Other pests of concern that farmers should be on the lookout for include grasshoppers, alfalfa weevils, and diamondback moths. Regular field scouting and monitoring of insect pest populations are important to determine if intervention is necessary.
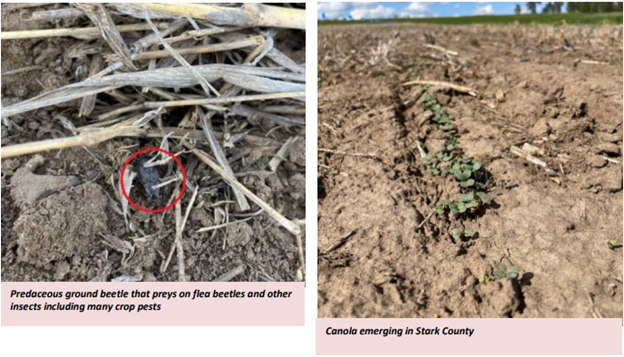
June 13, 2024
Southwest North Dakota
Most of the Southwest region has received some much welcome moisture over the course of last week, with the greatest amount falling in the Golden Valley County (0.63 inches). Even then, parts of Slope and Bowman Counties are still under Moderate Drought conditions.
Stripe rust disease in spring wheat has been identified in Slope County. Temperatures between 50-64°F coupled with intermittent rainfall are major factors favoring the disease. The best way to manage this disease is to plant resistant varieties.
Grasshopper and cereal aphid populations have been identified in most counties, however, with very low populations. We will keep an eye on those and see how these population levels develop.
Other than that, crops seem to be progressing well with wheat and barley anywhere between emerging and jointing stage, canola between emerging and 2 - 3 leaves, soybeans in the cotyledon stage and some sunflowers just emerging from the ground.
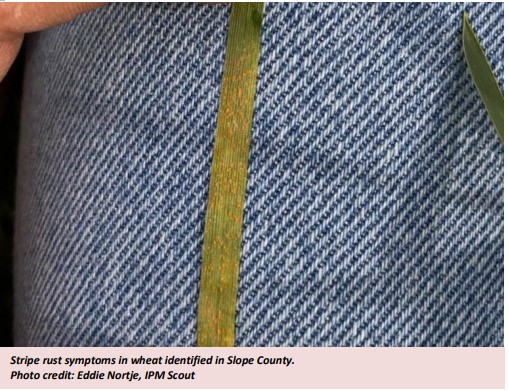
June 27, 2024
Southwest North Dakota
Over the last 7 days in Southwest North Dakota, total rainfall ranged from 0.24-inches in Dunn County to 1.86-inches in Morton County. After successive weeks of a dry spell, Bowman and Slope counties finally received over 1-inch of rainfall in the last 7 days. This much needed moisture is timely as the small grains and canola crops are entering the reproductive stages.
We’ve also seen a lot of active weather across the Southwest region of the state. Bowman was affected by a frost last week with temperatures dropping to 32°F. According to Penny Nester, ANR Ext. Agent in Bowman County, corn was the most affected crop, and the frost damage was most noticeable in low spots in the field and field margins. It is likely that the growing points were not affected but leaf injury was evident, so yield penalties may occur but not all is lost. No signs of frost damage on wheat or canola were reported in those areas.
There were reports of significant hail damage in Golden Valley, Slope and Adams Counties with isolated storms and about 1” hail reported south of Golva on Saturday evening. According to Ashley Ueckert, ANR Ext. Agent in Golden Valley, a few producers reported losing their fields. In central Slope County, Lillian McFadden, ANR Ext. Agent, also reported fields getting completely wiped out by hail in the central area of the County. The full extent of the damage is still being assessed. The general recommendation at this moment is to give it 7-10 days to give crops a chance to recover before assessing the damage and making any management decisions.
Regarding diseases, the weather conditions in the area, have increased the scab risk for susceptible wheat varieties to medium or high in all of Southwest NoDak (Figure 1). With some of the earlier planted spring wheat now well into the heading stage and going into flowering, timely fungicide applications are crucial to ensure good yields.
Insect populations remain fairly low but some relevant pests have just made their first appearance in our traps. Diamondback moth and wheat stem sawfly were present in Stark, Dunn and Billings Counties although in very low populations. True armyworm moths have been captured in greater numbers in wheat, but leaf damage is still low. Regular scouting to monitor pest populations is crucial before deciding whether or not to spray a pesticide.
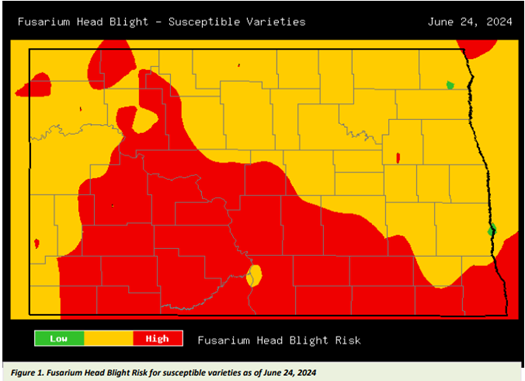
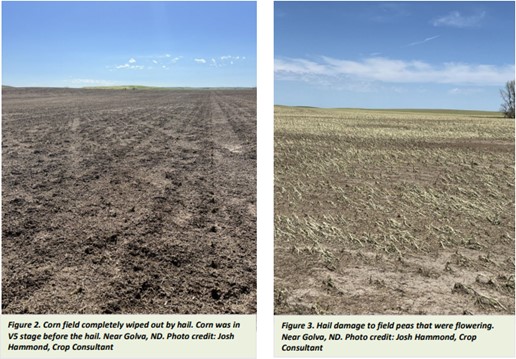
July 4, 2024
Southwest North Dakota
Another week of very active weather in Southwest North Dakota. Last week’s storm brought peak wind gusts at 10 ft of around 67 mph and scattered hail, with Fairfield in Billings County and New England in Hettinger County getting hit the hardest. Reports indicate 1.5 inches of hail in Fairfield, ND, causing significant crop damage (Figures 1 and 2). The wind gusts led to lodging in a few trials at the Dickinson Research/Extension Center, but the plants are expected to recover.
Total rainfall for the last week ranged from 0.05 inches in Bowman to almost 3 inches in Billings County, most of which fell in just one day. Parts of Bowman, Slope, and Golden Valley counties continue to experience moderate drought conditions.
There has been an increase in disease sightings during scouting trips, including loose smut and tan spot in wheat and covered smut in barley (Mercer County). Cool and wet conditions have kept pest populations relatively low, although stem sawfly numbers are steadily increasing.
Overall, crops are progressing well. However, the cool and wet weather has caused some farmers to report that their corn and soybeans are lagging due to a lack of growing degree units. In contrast, small grains have benefited from these cool weather conditions
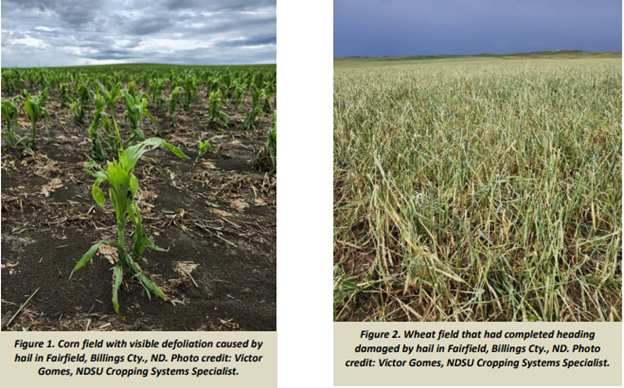
July 11, 2024
Southwest North Dakota
Over the last 7 days, we had some rainfall recorded in the Southwest, with the greatest precipitation in Sioux County (1.46 inches). However, a considerable portion of the area south of I-94 is still either abnormally dry or under moderate drought conditions, according to the U.S. Drought Monitor.
Concerning the crop progress, the winter wheat fields in the region are turning color, and spring wheat fields range from booting to flowering. Canola fields continue to bloom, turning the landscape a bright yellow. Soybeans are still a little behind, between VC and V3 stages, but hopefully, they will start to pick up with the increase in temperatures expected this month.
This week, we had our agronomy field day in Dickinson, where we covered topics including herbicide-resistant kochia management, lime recipe, cover crops management, fertilizer management for soybean and canola, a sprayer drone demonstration, and a NDAWN demonstration. Field days at the RECs will continue throughout this week and the following one. With the breadth of decisions to be made in agriculture, be sure to take advantage of these events to stay up-to-date with state-of-the-art research-based information and to make informed decisions.
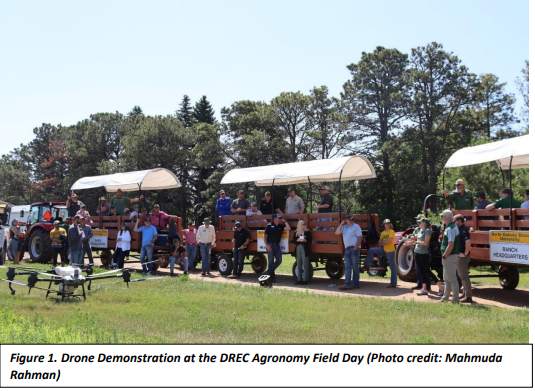
July 18, 2024
Southwest North Dakota
Over the last 7 days, very little rainfall was recorded in Southwest ND, with the greatest precipitation in Werner, Dunn County (0.76 inches). A storm occurred on July 13th near Cedar Lake in Slope Co., with 85 mph wind gusts, but except for some lodged plants, no major crop damage was reported. Besides that, temperatures rose well into the upper ranges in parts of the Southwest last week. A considerable portion of the area south of I-94 remains either abnormally dry or under moderate drought conditions, with reports of crops struggling in Bowman County.
Concerning crop progress, winter wheat fields in the region continue to turn color, and spring wheat fields range from booting to kernel water ripe stages. Canola fields are finishing blooming with some fields going into podding. With the increase in temperatures, soybeans have entered the reproductive stages, and many fields are flowering. Most field peas are at full pod and will begin to mature soon.
Spraying for small grain head diseases has been intense over the last couple of weeks. Despite these efforts, scab disease has been spotted at low levels across Southwest ND. Stripe rust remains the prevalent disease this growing season, with incidence levels of up to 70% reported last week in Slope County.
July 25, 2024
Southwest North Dakota
Dry conditions in Southwest North Dakota persist, with very little precipitation recorded over the last 7 days. The highest rainfall was registered in Golden Valley County at the Sentinel Butte station (0.53 inches). According to the National Integrated Drought Information System (NIDIS), the forecast indicates continued dry conditions over the next 7 days. These dry conditions, coupled with warm days, have been challenging for field crops.
In terms of crop progress, winter wheat fields are approaching harvest, while spring wheat fields range from heading to late milk stages. Canola fields are podding and filling grain. Soybeans continue blooming, with some fields entering the pod development stage. Field peas are maturing and will be ready to harvest soon.
Some insect populations have been increasing steadily in our area, especially Hessian fly and banded sunflower moth. Other than that, most insect pests of economic interest remain fairly low. Diseases in small grains are appearing in larger numbers, with scab being of particular concern.
August 1, 2024
Southwest North Dakota
Dry and hot conditions were observed in Southwest North Dakota over the last seven days, with maximum temperatures reaching the 100s F last week. Only the southern part of Grant County has seen significant precipitation, with 1.46 inches recorded at the Pretty Rock NDAWN station. Warm and dry conditions are expected to persist during the next seven days. Under these sustained conditions, some crops have been struggling, notably some corn fields showing signs of sunscald (Fig.1). Sustained drought conditions for more than four consecutive days during the vegetative stages of corn can lead to yield penalties of 1-3% per day. During the reproductive stages, the yield penalty can be as high as 3-9% per day.
There has been a lot of activity in the field over the last 7 days: winter wheat and barley are being harvested and baled, and the harvest for field peas has also begun. Spring wheat in the area ranges from finishing flowering to the soft dough stage. Canola is continuing to ripen but remains mostly green. Soybean fields are flowering and setting pods. Sunflowers are between the R1 and R3 stages, with some fields likely to see flowerheads opening soon. Some corn fields have just entered the tasseling stages with many others still lagging behind.
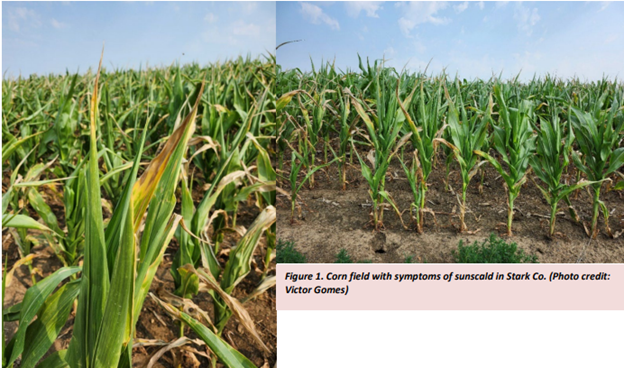
August 8, 2024
Southwest North Dakota
Dry and hot conditions in Southwest North Dakota have helped accelerate crop development, with most spring wheat changing color and nearing harvest. These conditions have also slowed disease progress in small grains, resulting in lower incidence and severity of scab and other head diseases across the region. Insect pest activity has decreased considerably for most species, except for grasshoppers, which had been steadily increasing their activity, extending beyond field edges and into the fields. However, with the anticipated drop in temperatures and increase in rainfall this week, grasshopper activity is expected to decrease going forward.
Preliminary results from our winter wheat variety trials show an average yield of 75 bushels per acre. More information will follow soon.
This week's rains will aid struggling corn fields, especially the earlier planted ones currently undergoing seed fill. However, most fields experienced water stress during critical stages for pollination, ear size, and seed number set, likely leading to reduced yields and abnormal ear formation.
Soybeans in the area are in various stages, from early flowering to the beginning of seed fill. Canola continues to ripen, with seeds starting to change color in the lower pods. Sunflowers are in different stages, with some fields almost at inflorescence opening and others well into flowering.
August 15, 2024
Southwest North Dakota
The first two weeks of August have brought some rain that will help get the crops going until the end of the season. Over the last 7 days, rainfall totals in Southwest North Dakota ranged from 0.02 inches in Sioux County and 0.86 inches in Dunn County, with more rainfall expected for this week. Regardless, the conditions in at least half of the Southwestern corner are either abnormally dry or moderate drought.
Harvest is progressing well in the area with some early planted spring wheat fields getting harvested. Preliminary reports account for average wheat yields in Bowman County (35-40 bushels/acre). Soybean fields are anywhere between flowering and seed fill. Canola fields have completed turning color and are drying out with seeds turning color in the pods. Sunflower fields are blooming with at least 50% of the floral heads open.
August 29, 2024
Southwest North Dakota
The last 7 days have brought much-needed moisture to our area, with the greatest precipitation recorded in Beach (1.64 inches). Temperatures were in the upper 80s and lower 90s for several days last week. Despite the recent rains, conditions in 50% of our area remain either abnormally dry or under moderate drought. Harvest activity was noticeable in areas that did not receive excessive moisture, while others are hopeful for a break in the weather to harvest without losing too much grain quality.
Some of the preliminary harvest reports include:
Stark and Billings Counties:
* West of Dickinson (Western Stark County and Billings County):
- Yields: Highly variable and spotty.
- Spring Wheat: Yields range from 30 to 60 bushels per acre, with reports of low test weights, shriveled kernels, and low protein levels.
- Other Crops: A stark contrast in yields, with one producer reporting 17-bushel durum while a nearby barley field yielded 95 bushels per acre.
- Environmental Impact: Drought and hail have significantly affected crops in this area.
* East of Dickinson and South of Richardton:
- Yields: Crops in this region are performing very well, benefiting from timely rains.
* Southern Stark County (Near New England):
- Yields: Also variable but generally above average.
- Spring Wheat: Low test weights have been noted, but some protein levels are as high as 15%.
Golden Valley County:
- Spring Wheat: Yields are reported at 20–30 bushels per acre, about one-third to one-half of expected outcomes. Some fields yielded up to 50 bushels per acre but with low test weight.
- Winter Wheat: Yields are within the normal range, averaging around 70 bushels per acre.
- Corn: Concerns are rising about the corn yield, because of how badly it was affected by drought and how delayed the crop development is, with some considering converting the crop to silage.
Mercer County:
- Harvest Progress: Slow due to unfavorable weather conditions, including high humidity, cool temperatures, and spotty rain showers.
- Barley: Yields are around 80 bushels per acre.
- Wheat: Yields range from 50 to 60 bushels per acre, with good quality, though some fields show slightly high falling numbers.
- Peas: Performing well, with many reports exceeding 40 bushels per acre.
- Corn: About two weeks behind schedule; an early frost could be damaging.
- Soybeans: Conditions have improved with recent rains, and the crop is starting to look better after a dry spell.
- Sunflowers: Just starting to flower, with a promising outlook.
- Canola: Some fields have been cut, but harvest is not yet widespread. Overall, yields are expected to be above average, contingent on improved weather for timely harvest.
Oliver County:
- Winter Wheat: Exceptional yields reported as the region benefited from timely rains throughout the season, with one farmer harvesting 800 acres averaging in the mid-90s bushels per acre.
- Spring Wheat: Yields mostly range from the upper 70s to low 80s bushels per acre.
- Quality: An exceptional small grain year overall, with several reports of ergot, though not at levels significant enough to cause rejections.
Huge thanks to the County Extension Agents Ashley Ueckert, Rick Schmidt, Craig Askim and Kurt Froelich for providing the data for this publication.
Planting cover crops still beneficial in fall, even under dry conditions
Victor Gomes
Extension Cropping Systems Specialist
701-456-1102, victor.gomes@ndsu.edu
Farmers will still be able to reap the benefits of having a cover crop emerge early in the spring even if they do not see any visible growth in the fall.
(Click the image below to view a high-resolution image that can be downloaded)
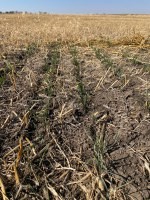
Appropriate cover crops like cereal rye, winter camelina, hairy vetch, triticale and winter wheat are winter hardy and have better chances of surviving the winter dormant in the soil and then emerging to protect the soil in the spring. (NDSU photo)
Many farmers in western North Dakota have planted cover crops like cereal rye or triticale this fall, but as dry as this season has been, many of the plants have yet to emerge, say North Dakota State University Extension specialists.
According to the North Dakota Agricultural Weather Network, much of western North Dakota has seen more than 30 days with less than one-half inch of rain, and some areas in the northwestern region of the state have had no significant rain in about four months. Under the current drought conditions, cover crops and winter cereals have been struggling to take in moisture and germinate.
“Even cover crops that were drilled or disked in, and benefited from the seed-to-soil contact, will have poor emergence if soil moisture is not adequate,” says Victor Gomes, NDSU Extension cropping systems specialist at the Dickinson Research Extension Center. “However, most cover crops have the ability to sit dormant in the soil over winter and germinate early in the spring, once they get enough warmth and moisture.”
This process is called dormant seeding and is different from frost seeding, which happens late in the winter after the soil freezes.
“One of the concerns that often comes up when cover crop seedlings do not emerge in the fall is that the “dormancy” won’t be broken in due time with moisture imbibition by the seed, and therefore the seed will die in the ground over winter,” says Chandler Gruener, NDSU Extension soil health specialist at the North Central Research Extension Center near Minot, North Dakota. “In this case, no germination is better than some germination.
If there is enough soil moisture (light rain) to promote seed sprouting but not enough moisture for further seedling growth, the crop will not survive the winter. For successful dormant seeding, farmers should plant seeds after the ground has cooled (35 degrees Fahrenheit) but before it freezes solid or is covered in snow for the winter, recommend the specialists.
It is important to bear in mind, however, that these winter cover crop species need to undergo a process called vernalization to be able to flower and produce seeds in the spring, says Gomes. Vernalization is the process where plants need to sense exposure to cool temperatures in order to reach flowering. To vernalize, seeds must have germinated prior to the cold period. Without vernalization, plants will show vegetative development only, producing only leaves. Dormant seeding is not recommended if the goal is seed production, as there is a risk of vernalization not occurring at the right time.
If soil temperatures stay below 35 degrees Fahrenheit or the moisture levels are insufficient to start germination, the seed's activity will stay low, preventing it from germinating. Germination kicks in when the soil temperature rises to around 38 to 40 degrees Fahrenheit and enough moisture is present.
Farmers will still be able to reap the benefits of having a cover crop emerge early in the spring even if they do not see any visible growth in the fall. Nevertheless, a few management practices should be considered:
- Cover crop choice is crucial for successful emergence in the spring. Appropriate cover crops like cereal rye, winter camelina, hairy vetch, triticale and winter wheat are winter hardy and have better chances of surviving the winter dormant in the soil and then emerging to protect the soil in the spring. Farmers should determine what to use as their cash crop. The recommendation is to never use cereal rye before a wheat or barley crop.
- It is important to incorporate seeds into the ground, not broadcast them. If the goal is improving soil health, then simply getting the seed in the ground is the most important factor to consider. Make sure seeds have good soil contact by drilling, not broadcasting, to avoid losing them to snow runoff.
- Termination timing is important. Because dormant seeded cover crops will have no growth in the fall, letting them grow as long as possible in the spring will ensure farmers get the benefits they are seeking. If planting a summer crop like corn or soybeans, the cover crops will likely benefit from a longer growth period. If planting a spring crop, like a small grain cereal, it is likely that the cover crops will not have much time to produce biomass. Still, some ground cover early in the spring is better than no ground cover. Overall, the recommendation is to terminate the cover crop 10 to 14 days before planting the cash crop.
- Planting green is another choice for termination timing that is adequate when lower biomass is present. This practice consists of planting the cash crop into a living cover crop. If this is done, it is best to terminate within a few weeks after planting to help prevent soil moisture from tying up. When terminating, the selection of the right chemical product is key to make sure the cover crop is effectively killed. It is recommended to follow the product and adjuvant guidelines required to ensure the cover crop is fully terminated.
“All things considered, dormant seeding still represents a risky choice and like any other planting event it takes careful planning and attention to weather patterns,” says Gomes. “While good cover crop emergence and establishment in the fall is preferred, when not enough moisture is available, having some ground cover available early in the spring is still better than no ground cover, and could help with soil dry-down, allowing for an earlier planting.
New program offers incentives for adoption of cover crops
Rutendo Nyamusamba, Extension Conservation Agronomist Specialist
Farmers for Soil Health is a farmer-driven initiative for farmers aimed at improving soil health through the adoption of cover crops. The collaboration among the Soy Checkoff, Pork Checkoff and National Corn Growers Association in partnership with state commodity groups and conservation organizations seeks to double the adoption of cover crops to reach 30 million acres by 2030.
Through the Farmers for Soil Health program, enrolled farmers receive incentive payments for adopting cover crops on their farms. The incentives are supported by a $95 million U.S. Department of Agriculture Partnerships for Climate-Smart Commodities grant.
North Dakota State University is playing a pivotal role in the partnership by providing science-based technical assistance and outreach to farmers wishing to enroll.
Helping to coordinate Farmers for Soil Health activities in North Dakota is Rutendo Nyamusamba, NDSU Extension conservation agronomist stationed at the Dickinson Research Extension Center.
“Cover crops have many benefits, including soil water and nutrient retention, reduced soil erosion, extending grazing periods and managing soil health aspects,” says Nyamusamba. “Our goal is to enroll 35,000 acres, or approximately 98 farmers into the program in North Dakota.”
“This program is unique because it was built by farmers for farmers, and it assists with the cost and learning curve of adopting cover crops,” says Ben West, Farmers for Soil Health executive director. “We built flexibility into the timing, allowing farmers to enroll their fields before or after planting cover crops.”
Enrollment is a simple online process that also positions farmers to take advantage of the new marketplace, providing a direct channel between farmers and end users. The fiscal year for the program is March 1 to February 28. The turnover between the previous year’s cover crop and the current year’s cover crops is February 28. The program also provides dedicated technical advisors to offer guidance and answer questions about transitioning fields to successful cover crop adoption.
If a field was planted to cover crops for the first time in the fall of 2024, it is eligible for a three-year contract payment of $50 per acre. This is structured as $25 the first year, $15 the second year and $10 the third year.
Visit FarmersforSoilHealth.com for information on eligibility and how to enroll online for cover crop payments.
NDSU Agriculture Communication – Nov. 13, 2024
Source: Rutendo Nyamusamba, 701-456-1113, rutendo.nyamusamba@ndsu.edu
Editor: Kelli Anderson, 701-205-9764, kelli.c.anderson@ndsu.edu
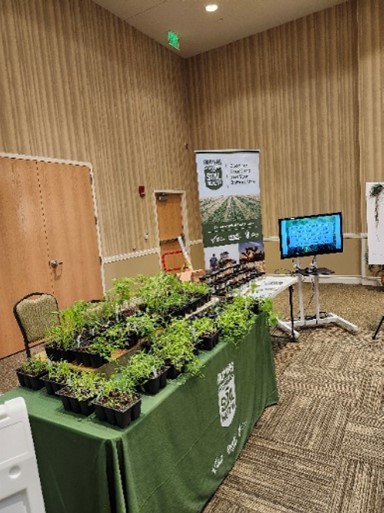
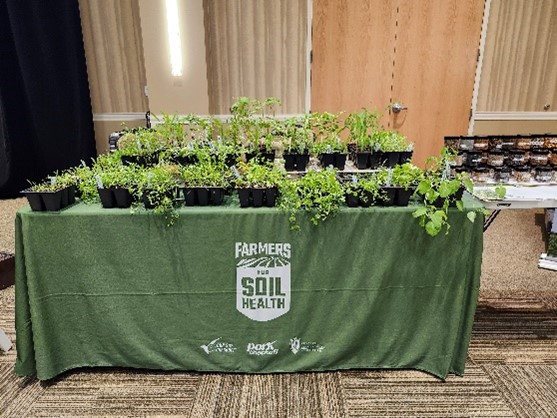
Sulfur Fertilizer for Canola Production in Southwest North Dakota
Krishna Katuwal
Research Agronomist
Dickinson Research Extension Center
701-456-1112
Introduction
Sulfur is the fourth most important macronutrient after Nitrogen, Phosphorous and Potassium for crop growth and development. Sulfur is a component of amino acids such as cysteine and methionine that serve as building block of proteins and enzymes, essential for chlorophyll production and also driver of tolerance to biotic pests and abiotic stressors. By activating enzymes, notably acetyl-CoA carboxylase, it supports oil formation in oilseed crops like canola through its role in lipid metabolic pathways. Current NDSU sulfur recommendation is 20 lb/ac for canola production in regions south/west of the Missouri Coteau in North Dakota. However, this recommendation is based on research conducted decades ago in north/east of the Missouri Coteau which is cooler and wetter compared to south/west of the Coteau. Therefore, there is a need for more immediate and region-specific research for sulfur recommendation in canola to support its growing acreage in south/west North Dakota. The objectives of this project were to evaluate effects of different sulfur fertilizer sources and application rates in canola yield and quality in southwest North Dakota and assess combination of these sulfur sources and rates with different nitrogen management (rates) in canola yield and quality in the region.
Materials and Methods
This is a second-year follow-up of the trial which was initiated in 2023. Research was conducted in three different locations across southwest North Dakota: Dickinson, Minot and Hettinger. Canola was solid seeded in May and right after planting treatments were broadcasted. We evaluated three different treatments in this study. One of our treatments was sulfur fertilizer sources which included ammonium sulfate, gypsum and elemental sulfur. Our second treatment was sulfur fertilizer rates: 0, 10, 20 and 30 lb/ac and third treatment was nitrogen rates: 0, 100 and 150 lb/ac. Nitrogen rate of 0 indeed had nitrogen equivalent to 30 lb/ac ammonium sulfate supplemented with urea. Each treatment was replicated four times and individual plot size was 30×10 ft. Our experimental design was randomized complete block design in split-split-plot arrangement. When canola reached physiological maturity, middle 5 ft across the length of each plot was harvested using plot combine for seed yield and oil content. A commercial grain tester was used to assess seed moisture content and test weight. Seed yield was adjusted to standard 8.5% moisture content.
Results
This year results identified a significant three-way interaction among sulfur fertilizer sources, sulfur and nitrogen rates. In ammonium sulfate, 20 lb/ac sulfur consistently ranked the top for seed yield across all nitrogen rates (Fig. 1). In gypsum and elemental sulfur, 20 lb/ac sulfur again ranked the top in two out of three different nitrogen rates that were evaluated in this project. Application of 20 lb/ac sulfur should produce optimum canola seed yield regardless of different nitrogen management. Within sulfur rate of 20 lb/ac, more linear increase in seed yield was observed increasing nitrogen rates for ammonium sulfate type sulfur fertilizer source than other sources. Applying 20 lb/ac ammonium sulfate along with adequate nitrogen may be a suitable fertilizer management strategy for maximizing canola production in southwest North Dakota. However, there were some inconsistencies in results as data showed greater seed yield in case of gypsum and elemental sulfur type sulfur fertilizer sources when minimal nitrogen was applied. Results were also not consistent across the two years of the present study. Therefore, further research is imperative to establish definitive conclusions. We will continue this study in future to develop robust recommendation for sulfur fertilizer application in canola production in the southwest North Dakota.
Fig. 1. Canola seed yield affected by different sulfur rates (0, 10, 20 and 30 lb/ac), sulfur fertilizer sources (Ammonium sulfate, gypsum and elemental sulfur) and nitrogen rates (0, 100 and 150 lb/ac). Columns marked with same letter are not significantly different at P ≤ 0.05.
Livestock Research
Integrated Systems Single Timed-AI Heifer Development and Non-Pregnant Heifer Finishing Profitability
Douglas G Landblom1, Songul Senturklu1,2, Lauren Hanna3, Bryon Parman4,
George Perry5, Steve Paisley6
1North Dakota State University, Dickinson Research Extension Center, Dickinson, ND
2Canakkale Onsekiz Mart University, Canakkale, Turkey
3North Dakota State University, Animal Science Department, Fargo, ND
4North Dakota State University, Department of Agribusiness and Applied Economics, Fargo, ND
5Texas A & M University, Agrilife Research, Overton, TX
6University of Wyoming, Sustainable Agricultural Research Extension Center, Lingle, WY
Research Brief:
Reproduction is the most profitable single management tool for the beef cattle enterprise. Heifers that become pregnant at the first service and calve early within the first 21 days of the calving season produce more pounds of beef compared to their later calving counterparts and have a greater probability of becoming pregnant early in subsequent breeding seasons (Burris and Priode, 1958). Moreover, heifers that calve early with their first calf have greater herd longevity and lifetime productivity compared to those that calve in the second 21 days or later (Cushman et al., 2013). In addition to the importance of pregnancy and early calving, accessing elite sires artificially ensures that replacement heifers have above average production opportunity due to genomic enhanced genetic potential.
Integrated crop-livestock beef cattle systems research at the NDSU-Dickinson Research Extension Center (DREC), has shown that regardless of steer frame score extended grazing of perennial and annual forages and delayed feedlot entry supported comparable meat quality and was consistently more profitable than feedlot control steers (Senturklu et al., 2018; 2019). This previous yearling steer grazing performance would be nutritionally supportive and is an ideal management system for replacement heifer development.
First service TAI using a 14-day Controlled Internal Drug Release® (CIDR-PGF2α-GnRH) program results in consistent pregnancy rates of 50-60% (Perry et al., 2012, 2015). A principal result from the use of progesterone delivered from a CIDR is to manipulate follicular waves by preventing the negative effect of premature PGF2α release from the uterus on corpus luteum survival (Patterson et al., 2019). For virgin heifers, a 14-d CIDR followed by PGF2α 16 d after CIDR removal and GnRH at the time of insemination 66 hr.±2hr after PGF2α has been a cost-effective timed artificial insemination (TAI) program. Moreover, using a single-TAI procedure produces heifers that are timed to calve early and the cost for keeping easy-calving heifer bulls year-around is eliminated.
Treatments:
1. Control (CTL): Drylot confinement during Single TAI breeding followed by grazing native range with clean-up bulls.
2. Native Range (NR): Following synchronized Single TAI, no clean-up bulls, after 85-d pregnancy determination non-pregnant heifers are finished and harvested at a federally inspected abattoir.
3. Annual Forage in an Integrated Cropping System + Native Range: Following synchronized Single TAI, no clean-up bulls, after 85-d pregnancy determination non-pregnant heifers are finished and harvested at a federally inspected abattoir.
Results:
A third year of data collection has been completed, summarized, and analyzed. Reproductive performance is shown in Table 1, replacement heifer grazing performance is shown in Table 2, non-pregnant heifer finishing performance is shown in Table 3, carcass measurements are shown in Table 4, and an abbreviated economic summary is shown in Table 5.
Heifer first service pregnancy rate was similar when comparing the drylot control treatment to the two other grazing-based treatments and ranged from 50.0% for the control and native range treatments to 56.0% among the integrated annual forage system. Grazing growth during and following synchronized breeding was greatest among heifers grazing a combination of native ranged and annual forage crops (field pea-forage barley mix, unharvested corn, multi-specie cover crop) gaining 278 lbs. and averaged 1.85 lbs./day. Control heifers housed in drylot before and during the AI breeding season grazed native range from July 24 to November 2, 2023. Movement from drylot to native range has an initial negative effect on gain resulting in slower overall growth performance. Control heifers gained 140 lbs. (1.39 lbs./day) compared to the heifers that grazed native range that gained 210 lbs. (1.41 lbs./day).
Non-pregnant heifers were not sold as feeder heifers, but retained through final finishing and marketed with the income and expenses combined with the bred heifer income and expenses to arrive at a system net return. Through a cooperative agreement with the University of Wyoming, the heifers are grown and finished at the UW Sustainable Agriculture Research and Education Center, Lingle, WY, and harvested at the Cargill Meat Solutions packing plant, Ft. Morgan, CO. Finishing values are shown Table 3. Control heifers entered the feedlot weighing less than the IAF and NR treatment groups; however, due to compensatory growth the control heifers ending weight was similar to the other treatments. Feed/pound of gain was 9.84, 10.38, and 9.05 and feed cost/heifer was $395.35, $334.39, and $353.65 respectively, for the control, IAF, and NR heifers. Accounting for initial processing, yardage, and feed costs the feedlot cost/lb of gain was $1.00, $1.09, and $0.94 respectively, for the control, IAF, and NR.
Heifer carcass measurements are shown in Table 4. Except for numerical differences across the different variables, there was very little difference measured between the control and grazing systems compared. Quality grade was exceptional with all of the heifer carcasses grading Choice or better (Prime).
Economically, systems net returns were excellent for the third year of the investigation. Net returns are shown in Table 5. The control system in which heifers that did not settle to AI were bred with clean-up bulls and had the highest net return after accounting for expenses of $1,313.26, compared to $900.91 for the integrated system and $1,041.64 for the native range system. Expenses among the integrated annual forage system including farming, feedlot, and freight to the feedlot and packing plant contributed to the lower net return. Similarly, the native range system net return was reduced due to feedlot finishing as well as freight to the feedlot and packing plant, but no farming expense, which contributed to a greater net return compared to the integrated annual forage system.
Table 1. Single TAI Pregnancy Summary (Year 3)
|
Table 2. Replacement heifer grazing performance (Year 3)
1 CTL – Drylot Control, NR - Native Range; ANN – Grazing Sequence: Native Range, Field Pea-Barley, Unharvested Corn, Cover Crops, and Cover Crop Bales. 2 Grazing start date: June 5, 2023, End grazing date November 2, 2023. 3 CTL removed from drylot after synchronized breeding period and grazed native range 4 P-Value for Trt – Treatment 5 Means with P < 0.05 differ significantly. 6 Marbling score: 400 = small; 500 = modest; 600 moderate |
Table 3. Non-pregnant heifer finishing performance (Year 3).
1 NR - Native Range; ANN – Grazing sequence: Native Range, Field Pea-Barley, Unharvested Corn, Cover Crops, and Cover Crop Bales. 2 Average grazing start date each year: NR May - 4, Pea-Barley - July 16, Unharvested Corn – August 17, Cover Crop – October 18, Bale Grazing – November 1, End grazing and transfer to feedlot – December 15. 3 NR and ANN steers were removed from the respective NR and ANN grazing treatments and fed cover crop hay for 43.66 d before transfer to the University of Wyoming, SAREC feedlot, Lingle, Wyoming. 4 Trt – Treatment 5 Means with P < 0.05 differ significantly.
|
Table 4. Post-breeding non-pregnant heifer carcass measurements (Year 3).
1 CTL – Drylot Control, NR - Native Range; ANN – Grazing sequence of Native Range, Field Pea-Barley, Unharvested Corn, Cover Crops, Cover Crop Bales. 2 CTL, NR and ANN steers were removed from their respective grazing assignments and transferred to the University of Wyoming, SAREC feedlot, Lingle, Wyoming. 3 Trt – Treatment 4 Means with P < 0.05 differ significantly. 5 Marbling score: 400 = small; 500 = modest; 600 moderate.
|
Table 5. Single fixed-time expense, income, and net return (Year 3)
Literature Cited: Burris, M. J., and B. M. Priode. 1958. Effect of calving date on subsequent calving performance. J. Anim. Sci. 17:527-533. Doi:10.2527/jas1958. 173527x Cushman, R. A., L. K. Kill, R. N. Funston, E. M. Mousel, and G. A. Perry. 2013. Heifer calving date positively influences calf weaning weights through six parturitions. J. Anim. Sci. 91:4486-4491. Patterson, D. J., J. M. Thomas, J. W. C. Locke, E. R. Knickmeyer, R. C. Bonacker, and M. F. Smith. 2019. Control of estrus and ovulation in beef cows. Proceedings, Applied Reproductive Strategies in beef Cattle, August 20-21, 2019, Knoxville, TN. Perry, G. A., E. L. Larimore, G. G. Bridges, R. A. Cushman. 2012. Management strategies for improving lifetime reproductive success in beef heifers. Proceedings, Applied Reproductive Strategies in Beef Cattle, December 3-4, Sioux Falls, South Dakota. Senturklu, S, D. G. Landblom, R. Maddock, T. Petry, C. J. Wachenheim, and S. I. Paisley. 2018. Effect of yearling steer sequence grazing of perennial and annual forages in an integrated crop and livestock system on grazing performance, delayed feedlot entry, finishing performance, carcass measurements, and systems economics. J. Anim. Sci., Vol. 96(6):2204-2218. Senturklu, S., D. G. Landblom, and S. I. Paisley. 2019. Effect of cover crop bale feeding (grazing) after native range or annual forage on steer grazing and feedlot performance, carcass measurements and carcass value. North Dakota Beef Report #1938, pp 24-28.
|
The Value of Alfalfa for Nutrient Recycling in a Diverse Crop Rotation
Douglas Landblom1 and Songul Senturklu2
1NDSU - Dickinson Research Extension Center, Beef Cattle and Integrated Systems Specialist
2Animal Scientist, Canakkale Onsekiz Universities, Canakkale, Turkey
Alfalfa is one of the most nutrient dense forages for livestock production and due to the crop’s nutrient density it is a valuable feed resource for all classes of ruminant livestock and swine to some extent. Going back in history before nutrient ingredient analysis were very well defined, livestock were fed alfalfa, because unknown ingredients helped animals thrive. For example, although nearly all swine herds are raised in confinement today, when pigs were reared outdoors in non-confinement, alfalfa was an important ingredient due to the protein, energy, mineral, and vitamin content (vitamins A, D, and E), which had not been discovered. Alfalfa harvested at the mid-bloom stage of development has the following nutrient analysis: CP 22%, TDN 58%, NE for gain 0.68 Mcal/kg, Neutral Detergent Fiber 47.1%, Acid Detergent Fiber 37.6%, Calcium 1.47, Phosphorus 0.22, Magnesium 0.35, Vitamin A 46,000 IU/kg, Vitamin D 2,000 IU/kg, and Relative Feed Value 114.
The primary reason for growing alfalfa is for livestock feed. Once established, alfalfa plants have a tap root and many fine roots that grow as much as 7-9 feet deep in the soil profile. The deep root structure taps into water that escapes the shallow rooting zone of other crops. Depending on the amount of available soil moisture, alfalfa can be cut initially followed by from one to three additional cuttings resulting in as many as four cuttings per year. Most years in western North Dakota dryland alfalfa will produce one and perhaps two cuttings. Under irrigation four cuttings per year are common.
Soil acidity problems have developed over time and the use of acid-based fertilizers. According to Cihacek et al. (2021) soils that contain higher amounts of clay and soil organic matter have a greater potential to resist acidification. In other words, the soil chemistry does a better job of buffering acidity. However, soils that have less organic matter and clay, and greater amounts of sand become acidic more easily. Soil acidity has a negative effect on plant nutrient availability and microbial activity, although fungi are somewhat more tolerant to acidic soils.
Considering alfalfa nutrient analysis, including the crop in a diverse crop rotation has the potential to insulate soils from developing soil acidity problems due to buffering from calcium and magnesium in the residue. Recent analysis results of alfalfa forage, root crowns (top 3 inches), and roots collected from 3 to 24 inches are shown in Table 1. Cihacek (2024) reported calcium and magnesium content of various soil surface crop residues of annual and perennial crops.
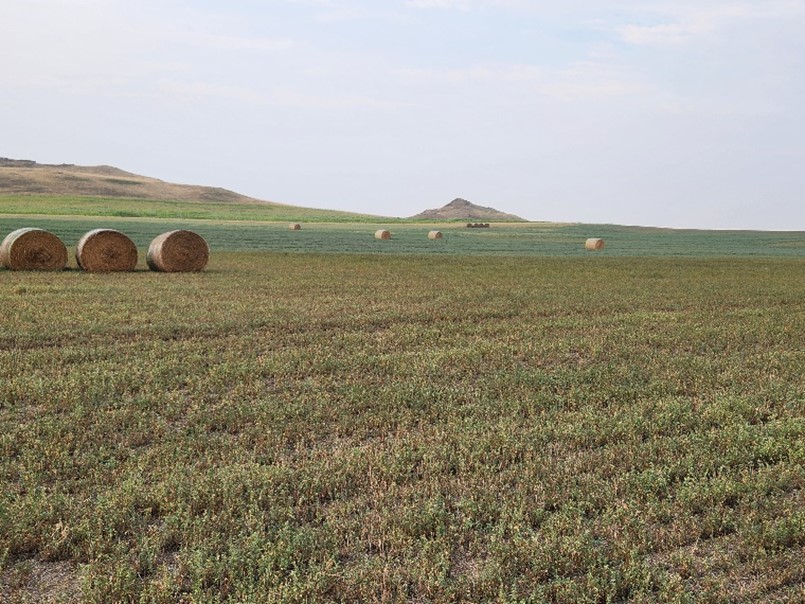
Table 1. Mid-bloom alfalfa forage, crown and root analysis.
|
Surface residue content was highest in soybean (1.26%), crambe (1.48%), and radish (1.88%). Landblom et al. (2024) in the pilot alfalfa investigation, measured the average root crown calcium content to be 0.95% and an average root crown weight of 31.64 grams. Using these two values and field alfalfa plant counts, it was determined that alfalfa crown residue after the crop has been terminated in a multi-crop rotation would provide a range of 5.8 to 9.4 tons of calcium per acre, which is significant with respect to maintaining a desirable soil pH balance in a multi-crop rotation. Although alfalfa is an extremely valuable cash crop for maintaining soil pH homeostasis in established alfalfa stands, establishing alfalfa in low pH soils is difficult. Unfortunately, in no-till farming systems remediating low pH soils it is best to apply lime at 2 to 4 tons per acre and incorporate with light discing. Since alfalfa fixes large amounts of N, the initial crop following alfalfa may not need nitrogen fertilizer; however, other fertilizer elements will need to be applied according to soil test.
References:
Cihacek, L., C. Augustin, R. Buetow, D. Landblom, R. Alghamdi, and S. Senturklu. 2021. What is Soil Acidity? NDSU Extension Bulletin SF2012, Fargo, ND, March 2021, pp 1-4.
Cihacek, L. Effect of Diverse Multi-Crop Rotations on Soil pH Balance. 2024 Soil Health Workshop Proceedings
Landblom, D., L. Cihacek, S. Senturklu. 2024. Alfalfa Value for Nutrient Recycling in a Diverse Crop Rotation. Dickinson Research and Extension annual report (In preparation).
Effect of Drought and Subsequent Precipitation (2016-2020) on Soil pH, Microbial Biomass, and Plant Nutrient Change in the Semi-Arid Region of Western North Dakota, USA
European Geoscience Union 24-7094
Douglas Landblom1, Songul Senturklu1,2, and Larry Cihacek3
(1) Dickinson Research Extension Center, North Dakota State University, Dickinson, North Dakota, USA, Department of Animal Science, (2) Canakkale Onsekiz Mart Universiteis, Canakkale, Turkey, (3) School of Natural Resource Sciences, North Dakota State University, Fargo, North Dakota, USA
Drought resulting from extended periods of limited precipitation have a substantial impact on soil pH, microbial biomass, and soil derived nutrients for plant growth. A long-term integrated crop, beef, and soil health research project at the Dickinson Research Extension Center is designed around a no-till diverse multi-crop rotation (spring wheat, cover crop, corn, pea-barley, sunflower). In this crop and animal production system, beef cattle graze the pea-barley, corn, and 13-specie cover crop to document microbial, fungal, and nutrient change over time and space. Precipitation during the first five-year crop rotation was normal to slightly above normal. However, the second five-year rotation was drier than normal resulting in nutrient concentration, reduced microbial biomass, and pH decline. Potential nitrogen mineralization of soil organic matter (SOM) in the crop rotation suggests that 8.4 mg N/kg of soil are mineralized for each 1.0% increase in SOM. The mean SOM content of soils in the study is 3.97%. For rain-fed crops, periods of reduced precipitation inhibit soil nutrient solubilization and translocation that negatively impacts a complex system of soil microbial respiration, fungal activity, plant nutrient supply, crop yield, and animal grazing days. The extent of soil drying in 2017 compared to moist soil in 2019 and somewhat drier soil in the 2020 cropping season will be presented. With drying, soil pH declined as soluble salt became more concentrated resulting in a more acidic condition. Naturally, reduced precipitation contributes to minimized plant and root growth, which contributed to reduced SOM content and nitrogen mineralization. For most of the crops in the diverse crop rotation, the percent of microbial active carbon, organic C : N ratio, and organic N : inorganic N ratio declined. Ward Lab Haney Test results for 24-hour microbial respiration provide measurements of microbial community and organismal diversity. Mean microbial biomass under drought conditions (2017), in the crop rotation, was 1,637 ng/g of soil. With the return to normal precipitation (2019) soil microbial biomass was 4,804 ng/g of soil; a 193.5% increase. While total microbial biomass increased with return to normal precipitation in 2019, arbuscular mycorrhizal fungi (AMF) did not reestablish in sunflower, cover crop, corn, and spring wheat-control, and only slight levels of AMF were measured in the pea-barley and spring wheat-rotation crops. Declining soil pH effects mineral nutrient availability among copper, manganese, zinc, and aluminum. At pH levels less than 5 (strongly acidic), aluminum availability becomes toxic to plants. Drought effected soil pH in this integrated systems research declined 9.5% to a mean crop pH value of 5.95, which at this pH level aluminum is sufficiently hydrated to be non-toxic. Return to normal precipitation (2019) increased the crop rotation pH mean to 6.58.
Integrated Systems Outreach Programs Prepared and Hosted by the
Dickinson Research Extension Center
Douglas Landblom1
1North Dakota State University, Dickinson Research Extension Center, Dickinson, ND
As a component of the integrated beef cattle and diverse cropping system research effort at the Dickinson Research Extension Center, programming to deliver information to interested stakeholders is an essential component of the Center’s mission.
The Soil Health Workshop has been a popular event for university students, farmers, and ranchers for many years. There is a plethora of topics related to soil health and the environment such that there is always new information to deliver to students and seasoned professionals alike. Adding to the Soil Health Workshop, the DREC planned and hosted the ND Chapter of the Soil and Water Conservation Society’s annual meeting educational session. The program was held at the Biesiot Activities Center and in the field utilizing the DREC’s integrated systems research laboratory located in Dunn County on Section 19-143-96.
In addition to soil health, cropping systems, and water conservation, the DREC hosted a beef cattle reproduction seminar. The Reproductive Strategies Seminar focused on heifer development, cow herd management, economics of the cattle price situation, and the market situation looking forward to 2025. This program was recorded on Zoom and can be accessed at:
Program agendas for the Soil Health Workshop, ND Chapter of the Soil and Water Conservation Society’s annual educational meeting, and the Reproductive Strategies seminar follow:
Dickinson Research Extension Center Soil Health Workshop set for Sept. 18
In addition to soil health presentations, multiple field stations will be available for viewing.
Roots, microbes, nutrient cycling, organic matter, and mycorrhizal fungi will be among the soil health topics discussed at a soil health workshop on Sept. 18, hosted by North Dakota State University’s Dickinson Research Extension Center (DREC).
The workshop begins at 8 a.m. and concludes at 2 p.m., MDT.
The morning session, held from 8 a.m. to 10:45 a.m., will be located at the Biesiot Activities Center (DSU Football Stadium), 398 State Avenue, Dickinson, North Dakota.
The morning topics and presenters are:
- Welcome-Douglas Landblom, DREC beef cattle and integrated systems specialist
- Soil is the foundation of civilizations: What is soil health & why are civilizations dependent on quality soils? - Susan Liebig, ND State Soil Quality Specialist, USDA-NRCS
- What are the 5 principles of the soil health and why are they important to agriculture sustainability? - Darrell Oswald, Burleigh County SCD
- Why is soil organic matter one of the most important soil components? - Naeem Kalwar, Soil Scientist, NDSU Extension Soil Health Specialist
- How does minimizing soil disturbance combined with a multi-crop rotation improve soil quality and nutrient cycling? -Hunter Ripplinger, Agronomist, USDA-NRCS
Lunch will be provided by Bravera Bank and Trust Grill Team, and then participants will caravan to the afternoon sessions at DREC Section 19, Integrated Systems Research Area, Manning, North Dakota. To drive to the site from Highway 22, turn west onto 15th St. SW and travel 5.2 miles to the field site.
The afternoon session will begin at 11:30 a.m. and conclude at 2 p.m. Topics and presenters are:
- Water movement through soil: the influence of roots, biopores, and soil structure-John Kempenich, Soil scientist, UDSA-NRCS and Krista Bryan, Soil Scientist, USDA-NRCS, Dickinson, MLRA Office
- The effect of a diverse multi-crop rotation on soil pH balance-Larry Cihacek, Soil Scientist, NDSU Natural Resource Sciences
Concurrent rotating field sessions will be available every 20 minutes. The stations and presenters are:
- The value of alfalfa for nutrient recycling in a diverse crop rotation-Doug Landblom, DREC Beef Cattle & Integrated Systems Specialist
- Soil texture, multi-specie cover crop, and water infiltration rate-Krishna Katuwal, DREC Research Agronomist
- Fungi: Why are mycorrhizal fungi associations with plant roots a valuable asset? – Danielle Harper, Asst. Prof. of Agriculture, DSU Agriculture and Technical Studies
- Crop rotation sequence using low and high water-use crops in the semi-arid region-Brent Gustafson, ND State Soil Health Specialist, USDA-NRCS
For meal planning purposes, please RSVP to Sheryl Schneider at 701-456-1120 or email Sheryl.schneider@ndsu.edu
For more information on the workshop, visit https://www.ndsu.edu/agriculture/ag-hub/research-extension-centers-recs/dickinson-rec/events/2024-soil-health-workshop
ND Soil & Water Conservation Society Annual Meeting Set for September 19
Practices that enhance carbon storage, drought and precipitation on soil microbiology, low soil pH on plant nutrient, and the value of alfalfa are the topics discussed at this annual meeting.
The workshop begins at 7:45 a.m. and concludes at 2:15 p.m. MDT.
This meeting will be held at Dickinson State University-Agriculture Auditorium, RM 102, 400 State Avenue, Dickinson, ND.
- Farming & Ranching Practices that Enhance Carbon Storage, Mike Gerbig, USDA-NRCS
- The Effects of Drought & Precipitation on Soil pH Microbiology, Douglas Landblom, DREC
- Break & Poster Session, All
- Ways ND Farmers are Applying Precision Agriculture Techniques on Their Farms: What are the Efficiencies? Jessica Kuntz & Andrina Turnquist, Agronomists, Gooseneck Equipment
- DSU Student Talk
- Effect of Low Soil pH on Plant Nutrient Availability and Aluminum Toxicity, Chris Augustin, DREC
- How is Soil Organic Matter Like Money in the Bank?, Carlos Pires, NDSU Soil Health Specialist
- Break and Poster Session, All
- DSU Student Talk
- What is the Value of Alfalfa in a Diverse Multi-Crop Rotation?, Marisol Berti, NDSU
- End of Technical Agenda
- Lunch and Awards Presentation
- Chapter Business Meeting
- Meeting Adjourn
DREC cattle reproductive strategies workshop set for Nov. 14
Heifer synchronization, heifer development, breeding season, capturing value for genetically superior cows, and single TAI will be among the topics discussed at a reproductive strategies workshop on Nov. 14, hosted by North Dakota State University’s Dickinson Research Extension Center (DREC).
The workshop begins at 9:00 a.m. and concludes at 3:15 p.m., MST, and will be emceed by Toby Stroh, Dickinson State University Department of Agriculture and Technical Studies.
The workshop will be held at Dickinson State University’s Agriculture Building Auditorium, Room 104,
400 State Ave., Dickinson, North Dakota.
The workshop agenda is:
- Registration
- Welcome and announcements - Douglas Landblom, DREC research specialist
- Managing cows for next year’s breeding season - Annette Steffan, Heart River Genetics, Belfield, North Dakota
- Reproductive management strategies to maximize production in natural service settings -
Joe C. Dalton, University of Idaho, Caldwell, ID (Zoom)
George A. Perry, Texas AgriLife Research & Extension Center, Overton, TX
- Lunch
- Capturing value for genetically superior calves - Troy Marshall, Dir. of Commercial Industry Relations, American Angus Association
- Nutritional management of replacement heifers for reproductive success - Shelby Rosasco, University of Wyoming, Laramie, WY
- Single-TAI, delayed feedlot entry, and economics research update and Beef producer heifer synchronization survey result update - Douglas Landblom, Dickinson Research Extension Center, Dickinson, ND, Songul Senturklu, Canakkale Onsekiz Mart University, Canakkale, Turkey
- Cattle price situation and other market outlook for 2024-2025 - Tim Petry, Extension Livestock Economist, Fargo, ND
- Closing comments and adjourn
Join Zoom Meeting
https://us02web.zoom.us/j/89341245299?pwd=yGav2G6fNLfLIXxyFzpBXS0ldO6b7r.1
Meeting ID: 846 2328 9323
Passcode: 604250
Please contact Douglas Landblom at 701-456-1109, douglas.landblom@ndsu.edu
DREC Outreach
Dickinson Research Extension Center Field Days Set for July 9 and 10
The North Dakota State University Dickinson Research Extension Center (DREC) will host a agronomy field day on July 9 and livestock-focused field day on July 10.
The July 9 event begins at 9 a.m. (MST) at the North Dakota State University Dickinson Research Extension Center. Lunch will be provided by the Dickinson Chamber of Commerce Ag Committee after the agronomy tour.
Presenters and topics for the agronomy tour are:
- Herbicide resistant kochia management-Joe Ikley, NDSU Extension Weed Specialist
- How much lime do my acid soils need?-Chris Augustin, DREC Director and Soil Scientist
- Data driven conservation-Lindsay Malone, NDSU Assistant Professor, Climate Smart Approaches in Ag
- Phosphorus management of soybeans-Dayne Tallier, Lindsay’s student
- Noxious weed management via drone-Josh Dipippo, Spotters Aerial Ag
- West river canola demands of sulfur-Krishna Katuwal, DREC Research Agronomist
- North Dakota Agriculture Weather Network Demonstration-Cassidy Holth, Meteorologist
The July 10 event begins at 9 a.m. (MST) at the Manning Ranch Headquarters in Manning, North Dakota and will conclude with a provided lunch.
Presenters and topics for the livestock tour are:
- Impact of heat stress in feedlot production systems-Rachael Gibbs, HREC Ruminant Systems Management Specialist
- Economics comparing drylot and grazing heifer development methods using fixed-time AI and finishing of non-pregnant heifers-Doug Landblom, DREC Associate Research Extension Center Specialist
- Selecting replacement heifers: Determining the objectives for expected positive herd change?-Lacey Quail, NCREC Extension Livestock Management Specialist
- Increasing carbon sequestration through grassland management-Llewellyn Manske, DREC Scientist of Rangeland Research
- Virtual Fence-Miranda Meehan, Associate Professor/Livestock Environmental Stewardship Specialist & Extension Disaster Education Coordinator
- Noxious weed management via drone-John Dipippo, Spotters Aerial Ag
- North Dakota Agriculture Weather Network Demonstration-Cassidy Holth, Meteorologist
For mor information, visit the DREC event page at NDSU.ag/DRECevents (https://www.ndsu.edu/agriculture/ag-hub/research-extension-centers-recs/dickinson-rec/events or contact Chris Augustin at 701-456-1103 or chris.augustin@ndsu.edu
The North Dakota State University Dickinson Research Extension Center (DREC) will host a cover crops field day set for September 10, 11, and 12.
The July 9 event begins at 9 a.m. (MST) at the North Dakota State University Dickinson Research Extension Center. Lunch will be provided. Please RSVP cristin.heidecker@ndsu.edu
5400 HWY 83 S |
1041 State Ave. |
|
Presenters and topics for the agronomy tour are:
Registration
Introduction + Farmers for soil health
How cover crops affect soil health?
Water relations in cover cropping systems
Water infiltration (tabletop demo)
Lunch
Current state of cover crops research tour
NRCS requirements and cost sharing
Outreach List 2024
Name | Topic | Location | Date |
Douglas Landblom | American Society of Animal Science: Beef Cattle Lactation Seminar | Online | 11-Jan-24 |
Douglas Landblom | Efficiency Reporting Payroll Certification Training | Online | 16-Jan-24 |
Douglas Landblom | Beef Cattle Improvement Association Annual Meeting Ramada Hotel | Bismarck, ND | 26-Jan-24 |
Douglas Landblom | Stark & Billings County Medora Beef Days | Medora, ND | 31-Jan-24 |
Llewellyn L. Manske | Zoom Interview: Use of twice-over management to improve the “green” aspects of meat as food with Forbs Mg writer Steve Savage | Zoom | 31-Jan-24 |
Douglas Landblom | Shark Farmer Integrated Systems Radio Interview | Mobile Phone | 8-Feb-24 |
Douglas Landblom | USDA/NIFA/Small & Medium-Sized Farms Annual Report Submitted | Online Portal | 13-Feb-24 |
Chris Augustin | Soybean fertility management | Stanley | 15-Feb-24 |
Chris Augustin | Soybean fertility management | Dickinson | 16-Feb-24 |
Krishna Katuwal | Western Soybean School Meeting Participation | Dickinson | 16-Feb-24 |
Douglas Landblom | Soil & Water Conservation Society February Monthly Meeting | Online | 20-Feb-24 |
Douglas Landblom | Team Development Program/CPR/First Aid Training | DREC Classroom | 21-Feb-24 |
Krishna Katuwal | Spring REC Conference Participation | Fargo | 28-Feb-24 |
Chris Augustin | ABC’s of acid soil management | Fargo | 6-Mar-24 |
Douglas Landblom | Soil & Water Conservation Society March Monthly Meeting | Online | 13-Mar-24 |
Llewellyn L. Manske | Farm Net News: Set target dates of cattle turnout for crested and native range on Red River Farm Network | Internet | 18-Mar-24 |
Douglas Landblom | European Geoscience Annual Meeting Present Abstract Poster | Vienna, Au | 13-20-Apr-24 |
Douglas Landblom | CattleFax Trends Webinar | Online | 22-Apr-24 |
Victor Gomes | Right of Way - Noxious Weed ID | Dickinson | 31-May-24 |
Chris Augustin | Fishing rod building class | Dickinson | 6,7,8-Jun-24 |
Douglas Landblom | ND Stockmen’s Region 5 Producer Meeting | Watford City, ND | 8-Jun-24 |
Llewellyn L. Manske | Attended: DREC Advisory Board Meeting | DREC ranch, Manning, ND | 11-Jun-24 |
Llewellyn L. Manske | Consulted: with Westervelt Ecological Service director Tyler Bell and field scientist Eveline Vega on “Management plan for the Blue Buttes Dakota skipper Conservation Bank” | McKenzie County, ND | 11-12-Jun-24 1, 10, 11-Jul-24 |
Douglas Landblom | DREC Advisory Board Meeting Research Project Update | DREC Ranch HQ | 11-Jun-24 |
Douglas Landblom | ND Stockmen’s Association Feedlot Tour | West/Central, ND | 18-Jun-24 |
Victor Gomes | DREC Field Day Radio Interview (KDIX) | Dickinson | 27-Jun-24 |
Llewellyn L. Manske | Field tour: with Westervelt Ecological Services field people to demonstrate the long-term problems from prescribed burns in western North Dakota | Western North Dakota | 3-Jul-24 |
Llewellyn L. Manske | Consulted: with Xerces Society members on the management to reduce grasshopper populations without pesticides | Dickinson, ND | 8-Jul-24 |
Llewellyn L. Manske | Attended: DREC Agronomy Field Day | Dickinson, ND | 9-Jul-24 |
Douglas Landblom | DREC Crop Field Day | Dickinson, ND | 9-Jul-24 |
Krishna Katuwal | Sulfur Fertilization in Canola (DREC field day-Agronomy) | Dickinson | 9-Jul-24 |
Chris Augustin | Acid soil lime recommendations | Dickinson | 10-Jul-24 |
Krishna Katuwal | DREC annual field day-Manning Ranch | Dickinson | 10-Jul-24 |
Llewellyn L. Manske | Presentation: Increasing carbon sequestration with grassland management. DREC Ranch Field Day. 15 min, 54 people | DREC ranch, Manning, ND | 10-Jul-23 |
Douglas Landblom | DREC Ranch Field Day | Ranch HQ | 10-Jul-24 |
Douglas Landblom | American Society of Animal Science Research Abstract Poster | Calgary, AB | 21-26-July-24 |
Victor Gomes | Abiotic Stress ID – NDSU Extension Professional Development Day | Minot | 30-Jul-24 |
Chris Augustin | State land judging | Rolla | 1-Aug-24 |
Victor Gomes | ND Soybean Growers Magazine Interview |
| 1-Aug-24 |
Douglas Landblom | USDA/NIFA/SMF Progress Report Submission | Online Portal | 8-Aug-24 |
Krishna Katuwal | Grant Writing Workshop | Bismarck | 9-Aug-24 |
Douglas Landblom | USDA/NIFA Project Review | Online Teams | 20-Aug-24 |
Victor Gomes | NDSU Ag Minute Podcast Interview (KFGO) |
| 21-Aug-24 |
Krishna Katuwal | Grant Writing Workshop | Bismarck | 23-Aug-24 |
Douglas Landblom | Beef Reproductive Task Force Annual Meeting | Athens, GA | 3-6-Sep-24 |
Victor Gomes | Radio Interview (KBJM) |
| 3-Sep-24 |
Victor Gomes | Radio Interview (KQXL) |
| 5-Sep-24 |
Krishna Katuwal | Fertility Management in Canola (NDSU All Ag Conference) | Fargo | 6-Sep-24 |
Victor Gomes | Water relations in cover cropping systems and species selection – Cover Crops Field Days | Minot, Dickinson and Williston | 9,10,11-Sep-24 |
Rutendo Nyamusamba | Attended the Minot Cover Crop Field Day | Minot | 10-Sep-24 |
Chris Augustin | Cover crops in western North Dakota and demonstration | Dickinson | 11-Sep-24 |
Llewellyn L. Manske | Lecture: How grasses grows, DSU Range Mgt Class. 1 hr, 12 people | Dickinson, ND | 11-Sep-24 |
Rutendo Nyamusamba | Attended the Dickinson Cover Crop Field Day | Dickinson | 11-Sep-24 |
Rutendo Nyamusamba | Attended the Williston Cover Crop Field Day | Williston | 12-Sep-24 |
Chris Augustin | Cover crops in western North Dakota and demonstration | Williston | 12-Sep-24 |
Llewellyn L. Manske | Field tour of ND Badlands: DSU Range Mgt Class 27 people | Badlands, ND | 16-17-Sep-24 |
Krishna Katuwal | Ryan Kadrmas Farm Field Day | Dickinson | 17-Sep-24 |
Krishna Katuwal | Cover Crop and Soil Texture (Soil Health Workshop) | Dickinson | 18-Sep-24 |
Douglas Landblom | DREC Soil Health Workshop Dunn County Sec 19-143-96 | DREC Ranch | 18-Sep-24 |
Rutendo Nyamusamba | Attended the DREC Section 19 Field Day | Manning | 18-Sep-24 |
Douglas Landblom | Soil & Water Conservation Society Annual Meeting Hosted by the DREC | DSU Auditorium 104 | 19-Sep-24 |
Victor Gomes | Small grain harvest nears completion with mixed results. Article for a group of local newspapers (GS Publishing) | Southwest North Dakota | 19-Sep-24 |
Krishna Katuwal | ND Soil & Water Conservation Society Annual Meeting | Dickinson | 19-Sep-24 |
Llewellyn L. Manske | Lecture: Biology of grazing management. DSU Range Mgt Class. 1 hr, 11 people | Dickinson, ND | 25-Sep-24 |
Chris Augustin | Lime recommendations | Fargo | 6-Nov-24 |
Douglas Landblom | NDSU All Ag Affairs Conference & Poster Session | Fargo, ND | 5-6-Nov-24 |
Rutendo Nyamusamba | All Ag Conference – Farmers for Soil Health Booth | Fargo | 06-Nov-24 |
Victor Gomes, Chandler Gruener | Planting cover crops still beneficial in fall, even under dry conditions. NDSU Extension Newsletter |
| 8-Nov-24 |
Victor Gomes | Radio Interview – Red River Farm Network | Grand Forks | 12-Nov-24 |
Krishna Katuwal | Canola Fertility Management (CSSA meeting) | San Antonio | 13-Nov-24 |
Chris Augustin | Dickinson Research Extension Center Opportunities | Fargo | 15-Nov-24 |
Douglas Landblom | DREC Reproductive Seminar: Heifer Development, Cow Herd Mgmt., and Economics | Dickinson, ND | 14-Nov-24 |
Douglas Landblom | Soil & Water Conservation Society December Meeting | Online | 05-Dec-24 |
Douglas Landblom | DREC Advisory Board Meeting Research Project Update | DREC Classroom | 12-Dec-24 |
Outreach Collaborators and Grants 2024
Collaborators | Grant Title | Granting Agency | Amount ($) |
Chris Augustin, Leandro Bortolon, John Rickertsen | Sulfur fertilizer impacts on hard red spring wheat grown in western North Dakota | ND Wheat Commission | 30,000 |
Chris Augustin, Leandro Bortolon, John Rickertsen | Sulfur Fertilizer Impacts on Canola Grown in Southwest North Dakota | Northern Canola Growers Association | 30,000 |
Leandro Bortolon, Chris Augustin | Variability of soybean yield related to soil acidity in Western ND | ND Soybean Council | 28,370 |
Leandro Bortolon, Chris Augustin | Soybeans interseeded with cover crops in Western North Dakota | ND Soybean Council | 27,370 |
Lindsay Malone, Chris Augustin, Leandro Bortolon, Szilvia Katalin Yuja | State-wide evaluation of P rates required for soybean in North Dakota | ND Soybean Council | 51,790 |
Jason Harmon, Torre Hovick, Miranda Meehan, Zachary Carlson, Ben Geumont, Kevin Sedivec, Chris Augustin, Travis Seaborn | Virtual fencing to support livestock production and conservation of grassland wildlife and invertebrates | Natural Resources Conservation Service | 1,092,382 |
Chris Augustin | Fishing rod building 4-H trunk | ND Game and fish Department | 3,215 |
Collaborators | Grant Title | Granting Agency | Amount ($) |
Carlos A.B. Pires, Joao Paulo C. Flores, Victor Gomes, Brady J. Goettl, Chandler Gruener | Dynamic Soil Properties Assessment and Digital Mapping Across Diverse Land Uses in the Northern Great Plains | NRCS | 494,120 |
Christopher L. Augustin, Krishna Katuwal, Victor E. de V. Gomes | Sustainable Soils for Healthy Communities and Climate Resilience in the Semi-Arid West | Agricultural Research Service | 200,000 |
Peer reviewed publications | |||
Gomes, V.E.V.; Lindsey, A.J.; Zarnstoff, Mark; Houx, James; Novais, Wanderson; Koirala, Nassib; Lee, Corinne. Defoliation level and timing affect popcorn yield traits in the Midwestern United States. Field Crops Research Journal, e109472. https://doi.org/10.1016/j.fcr.2024.109472 | |||
Gomes, V.E.V.; Kennedy, A.M.; Darcy, A.R.; Lindsey, A.J. (2024) Hormone type, soaking duration and seed coating affect dormancy and germination of pennycress (Thlaspi arvense L.) lines. Seed Science and Technology, 52(2), 163-175. https://doi.org/10.15258/sst.2024.52.2.03 | |||
Gomes, V.E.V.; Lindsey, A.J.; Novais, W., Thomison, P.R.; Geyer, A.B.; Roth, G.W.; Resse, K.D. (2024) Narrow row maize cultivation increased grain and forage yield of hybrids in Ohio and Pennsylvania. Agronomy Journal, 116(4), 1817-1827. https://doi.org/10.1002/agj2.21614 | |||
Gomes, V.E.V.; Mesquita, R.O.; Innecco, R.; Nunes, L.R. (2024) Agronomic performance of grain amaranth in the semi-arid region as a function of the planting arrangement. Caatinga Journal, 37, e11913. https://doi.org/10.1590/1983-a21252024v3711913rc |
2024 Outreach List for DREC
|
2024 Weekly Updates
Name | Topic | Date |
Chris Augustin | The Dickinson Research Extension Center team has been working to develop lime recommendations to help producers remediate and manage acid soils | 2-Jan-24 |
Doug Landblom | A Comparison of heifer development methods and synchronized timed artificial insemination | 9-Jan-24 |
Chris Augustin | Basics of sulfur management | 16-Jan-24 |
Llewellyn L. Manske | Increasing grassland soil organic carbon | 23-Jan-24 |
Chris Augustin | Cow herd software update | 30-Jan-24 |
Doug Landblom | 2023: Record number of non-pregnant open cows | 6-Feb-24 |
Chris Augustin | Fertilizer impacts on annual forage nitrates | 13-Feb-24 |
Llewellyn L. Manske | Spikemoss (Clubmoss) is a symptom of a soil functionality problem | 20-Feb-24 |
Chris Augustin | Dickinson Research Extension Center Annual Report | 27-Feb-24 |
Doug Landblom | Influence of vaccine type and administration timing on synchronized fixed-time AI reproductive performance in beef cattle | 5-Mar-24 |
Chris Augustin | Soybean breeding efforts at the Dickinson Research Extension Center | 12-Mar-24 |
Llewellyn L. Manske | Western wheatgrass tiller growth | 19-Mar-24 |
Chris Augustin | Dickinson Research Extension Center Field Days July 9 and 10 | 26-Mar-24 |
Doug Landblom | Controlling fly populations is economically important | 2-Apr-24 |
Chris Augustin | Fertilizer tests this spring | 9-Apr-24 |
Llewellyn L. Manske | Blue grama tiller growth | 16-Apr-24 |
Chris Augustin | The Dickinson Research Extension Center collaborates with various faculty at the NDSU main campus in Fargo | 23-Apr-24 |
Doug Landblom | Effects of drought and subsequent precipitation on soil pH, microbial biomass, and plant nutrient change | 30-Apr-24 |
Chris Augustin | NDAWN at DREC | 7-May-24 |
Llewellyn L. Manske | Needle and thread tiller growth | 14-May-24 |
Chris Augustin | DREC field days | 21-May-24 |
Doug Landblom | Natural resource programs coming in September | 28-May-24 |
Victor Gomes | IPM Crop survey program launches this week | 4-Jun-24 |
Llewellyn L. Manske | Yellow owlclover autecology | 11-Jun-24 |
Chris Augustin | 2024 DREC field days | 18-Jun-24 |
Doug Landblom | Cattle facial recognition | 25-Jun-24 |
Chris Augustin | 2024 DREC field days | 2-Jul-24 |
Chris Augustin | 2024 DREC field days | 8-Jul-24 |
Llewellyn L. Manske | White prairie aster autecology | 16-Jul-24 |
Victor Gomes | Accurately estimating crop yields | 23-Jul-24 |
Doug Landblom | Soil health workshop scheduled for September 18 | 30-Jul-24 |
Chris Augustin | New shop/laboratory facility is open | 6-Aug-24 |
Llewellyn L. Manske | Purple coneflower autecology | 13-Aug-24 |
Victor Gomes | Cover crops field days set for September 10, 11, and 12 | 20-Aug-24 |
Doug Landblom | Soil health workshop and soil and water conservation society annual meeting scheduled for September | 27-Aug-24 |
Chris Augustin | Cover crops field days set for September 10, 11, and 12 | 3-Sep-24 |
Llewellyn L. Manske | Prairie wildrose autecology | 10-Sep-24 |
Victor Gomes | Don’t let nitrates ‘feed’ you trouble: test forages before grazing or haying | 17-Sep-24 |
Doug Landblom | Is early weaning an option this fall | 24-Sep-24 |
Chris Augustin | Soil sampling trouble shooting | 2-Oct-24 |
Llewellyn L. Manske | Creeping juniper autecology | 8-Oct-24 |
Victor Gomes | Fall applied N fertilizer management | 15-Oct-24 |
Doug Landblom | Beef cattle reproductive strategies seminar | 22-Oct-24 |
Llewellyn L. Manske | Grass plant survival mechanisms | 30-Oct-24 |
Llewellyn L. Manske | Internal grass growth mechanisms | 5-Nov-24 |
Victor Gomes | Planted cover crops this fall but saw no emergence? Not all is lost | 12-Nov-24 |
Doug Landblom | Effect of drought and subsequent precipitation (2016-2020 on soil parameters following grazing | 19-Nov-24 |
Chris Augustin | Happy Thanksgiving! | 26-Nov-24 |
Weather Summary | ||||||
Max Temp | Monthly Avg. Temp | |||||
Month | 2024 | 2023 | 2022 | 2024 | 2023 | 2022 |
Jan | 22.0 | 26.9 | 23.0 | 13.6 | 19.1 | 12.7 |
Feb | 38.0 | 30.2 | 27.9 | 28.3 | 20.7 | 15.7 |
Mar | 37.5 | 25.2 | 40.1 | 25.5 | 16.7 | 29.3 |
Apr | 58.1 | 48.2 | 42.7 | 44.9 | 37.4 | 34.0 |
May | 66.4 | 71.6 | 63.6 | 52.6 | 57.8 | 51.3 |
Jun | 74.9 | 80.8 | 74.6 | 62.1 | 67.5 | 62.4 |
Jul | 86.5 | 81.5 | 84.0 | 72.1 | 68.5 | 70.6 |
Aug | 80.8 | 81.0 | 85.1 | 68.2 | 68.2 | 70.7 |
Sep | 82.9 | 75.8 | 78.2 | 65.9 | 62.0 | 63.6 |
Oct | 65.5 | 53.6 | 56.4 | 49.7 | 42.6 | 45.3 |
Nov | 38.5 | 53.5 | 30.9 | 28.5 | 32.6 | 22.2 |
Dec |
| 39.6 | 16.0 |
| 30.3 | 7.5 |
Precipitation | ||||||
Month | 2024 | 2023 | 2022 | |||
Jan | 0.23 | 0.33 |
| 0.15 | ||
Feb | 0.29 | 0.25 |
| 0.22 | ||
Mar | 0.57 | 1.78 |
| 0.48 | ||
Apr | 1.35 | 0.30 |
| 4.16 | ||
May | 2.35 | 2.69 |
| 3.17 | ||
Jun | 2.75 | 1.91 |
| 2.02 | ||
Jul | 0.88 | 2.21 |
| 3.71 | ||
Aug | 1.28 | 3.25 |
| 0.28 | ||
Sep | 0.12 | 1.32 |
| 0.93 | ||
Oct | 0.00 | 1.24 |
| 1.84 | ||
Nov | 0.15 | 0.02 |
| 1.51 | ||
Dec |
| 0.12 |
| 1.69 |
| |
Total |
|
| 15.42 |
| 20.16 |
|